What inspired your research into developing medical-grade wearable sensors?
We decided to develop this new class of devices mostly because of the gap in the capabilities of current wearable devices. Form factors have not changed for a long time and shortcomings, including the constant need for device interaction such as charging and lack in sensor fidelity often induced by bulky device construction, restrict the devices’ capabilities significantly.
We started this project to overcome these limitations to enable the seamless collection of biosignals and provide a platform for new sensors that are enabled by intimate integration with the body.
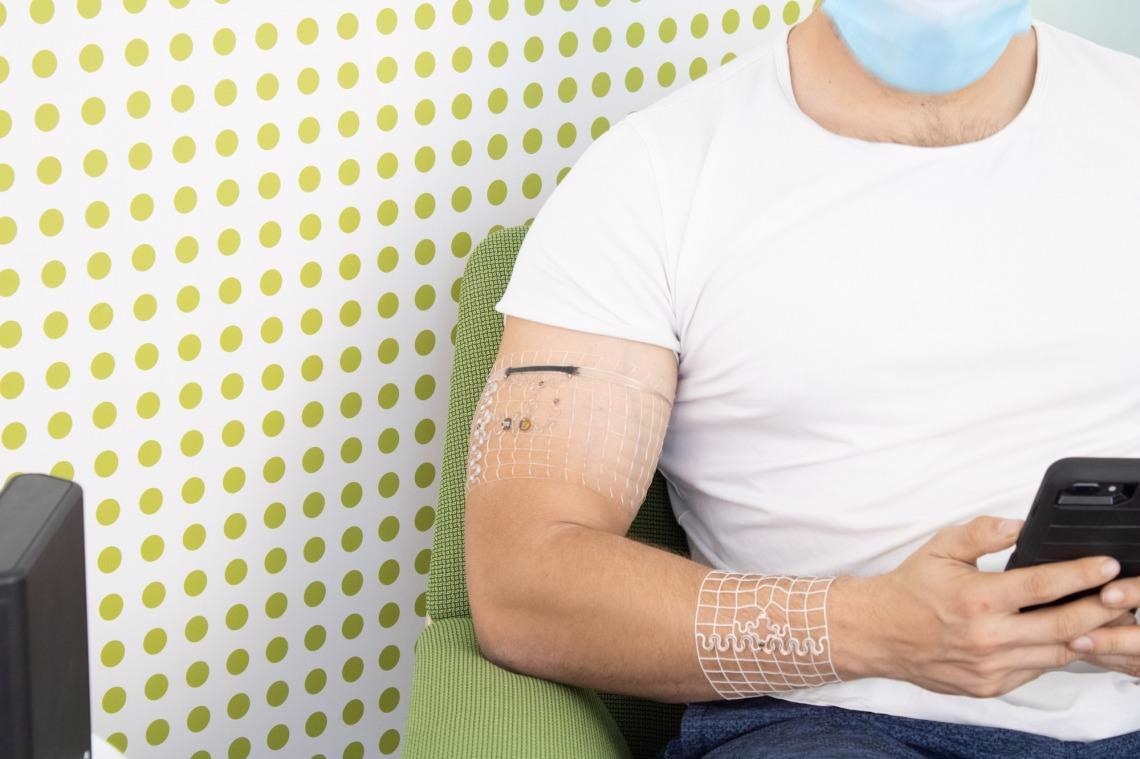
Image Credit: University of Arizona
You and your team have created a type of wearable sensor that is referred to as a ‘biosymbiotic device’. Could you give an insight into what this device is and how it works?
Biosymbiotic devices are soft electronics that are directly tailored to an individual to provide the best bio integration possible.
We solve the challenge by using 3D scans of the wearer to build a device that is unique to the individual. We have developed a technique to 3D print them, which has the benefit of being able to create unique devices and we can tailor sensor location to enable the best possible signal fidelity.
Our goal was also to create a system that is almost imperceptible to the subject wearing it, this is why we needed to eliminate bulky batteries. We achieved this by using a technique called power casting – a technique that recharges devices or even completely powers them in the proximity of this infrastructure. This means that users never have to recharge. In fact, as shown in our publication, devices stay topped up for weeks at a time.
What are the key benefits provided by this novel wearable sensor?
The key benefits of the system are derived from the personalized structure that can intimately conform to the wearer. This enables sensor placement in the most relevant locations to extract high fidelity biosignals, opening up avenues to capture more information than with conventional designs.
For example, we can integrate stretchable strain sensors that allow for the measurement of muscle activation by sensing the circumferential change, which is currently not possible with wearable devices.
We can also integrate low thermal mass temperature sensors which enable the measurement of milli Celsius fluctuations that are induced by exercise. This is not possible with large devices such as smartwatches because they have too much thermal mass.
Another key benefit is the wireless power transfer that enables 24/7 operation. This results in the ability to provide uninterrupted data streams where typical solutions have to be taken off to recharge. The combination of the stretchable mechanics and the continuous operation also combats user fatigue which results in better device acceptance.
In your research, you mentioned that there is nothing quite like this out there. Why have researchers never before attempted to create a device that is tailored directly to a person and that uses wireless power casting?
I think the reason why this was not attempted before is that some of the technologies were not available. Far field power casting that is now commercially available and is a fairly new development and the ability to 3D print elastomeric materials in an accessible way is also a recent development.The same applies for strategies for stretchable electronic circuits.
Our devices are a culmination of these novel techniques.
What are the current limitations of current wearable sensors, such as wearable patches that stick to the skin, smartwatches and ECG monitors, and how does your 3D-printed custom-fitted device overcome these limitations?
I have outlined some of the limitations in terms of sensors in my previous examples on temperature sensing where thermal mass of current devices is prohibitive, however one fundamental limitation for devices that are attached to the body using adhesives is the epidermal turnover of the skin, meaning the renewal of the skin. This turnover results in the renewal of the top layer of the skin every 5-7 days which poses a finite lifetime for adhesive based devices.
Epidermal turnover of the skin. This turnover results in the renewal of the top layer of the skin every 5-7 days. Image Credit: Shutterstock.com/ tofuneko
The limitations of wearable devices such as smartwatches are typically the high mass which causes motion artefacts and limits the electrical contact to the skin, resulting in lower fidelity.
Your sensor has the ability to specialize sensor placement. How is this advantageous to the user?
This is very beneficial for some sensor types, for example, if you would like to get something close to core body temperature we can make devices that place temperature sensors in the auxilia region (armpit) which provide a much more accurate reading than estimating this with a wristband based device.
We can also place new sensor types, such as our circumferential strain sensors, onto the area of the muscle with most deformation during exercise which provides an intimate insight into exercise intensity.
When testing the device’s ability to monitor parameters, including temperature and strain while a person jumped, what did you discover?
For biosymbiotic devices, the sensors have good contact with the skin and high fidelity bio data is able to be attained, even during extreme events. This advantage is immediately visible when compared to a classic wearable device that features a battery and strap attachment that is quite heavy.
During a jump, the mass of the device induces oscillations in the readouts that are not present on the actual limb. For our devices, that only weigh milligrams, this motion artefact is not present, highlighting a fundamental advantage. This will also be relevant for optical sensing techniques such as PPG and SPO2 measurements.
In terms of application, what industries will benefit from this biosymbiotic device?
The application of biosymbiotic devices can range anywhere from diagnostic devices that allow for at-home detection and management of disease to human performance-related applications that characterize training and recovery. Devices may also be used to monitor the environment around the wearer and provide feedback.
As the devices are personalized, individualized sensor configurations may also be possible to cover specific scenarios, for example, for comorbidities.
Did you come across any challenges during your research, and if so, how did you resolve them?
The integration of many new technologies always poses challenges, especially when creating devices that are very different from the current state of the art. Luckily, I have a team of very talented graduate and undergraduate students that are dedicated to pushing the envelope and are very motivated to find solutions to the technological challenges we encountered throughout our research and for translation to products that are highly scalable.
What does the future of wearable sensors look like to you?
I think we will see a lot more connected sensing solutions that make use of multimodal sensor systems that harness these data streams using artificially intelligent algorithms that will support the therapeutic and diagnostic decisions of clinicians.
Devices will also be used much more extensively for personalized health, meaning personalized training for athletes, personalized recommendations for diet and lifestyle and sensing our surroundings to optimize performance. This may also help with public health.
What are the next steps for your research?
We are currently expanding the capabilities for biosymbiotic devices and exploring new sensing possibilities that are enabled by the platform. We are also performing clinical trials with devices to benchmark their performance against the gold standard to evaluate their use in automated at-home diagnostics.
Where can our readers find out more about this novel wearable sensor?
Readers can find out more information on this work in our recently published paper which is open access. More information on the team is also available on our research group's homepage.
About Dr. Philipp Gutruf
Dr. Philipp Gutruf is an Assistant Professor in the Biomedical Engineering Department and Craig M. Berge Faculty Fellow at the University of Arizona. He received his postdoctoral training in the John A Rogers Research Group at Northwestern University and received his PhD in 2016 at RMIT University (Australia).
His research group focuses on creating devices that intimately integrate with biological systems by combining innovations in soft materials, photonics and electronics to create systems with a broad impact on health diagnostics, therapeutics and exploratory neuroscience. In the last 5 years he has authored over 50 peer-reviewed journal articles, received 5 patents and his work has been highlighted on 8 journal covers. More recently his group's work is featured in journals such as Nature Communications, Science Advances, PNAS and Nature Biomedical engineering.
Disclaimer: The views expressed here are those of the interviewee and do not necessarily represent the views of AZoM.com Limited (T/A) AZoNetwork, the owner and operator of this website. This disclaimer forms part of the Terms and Conditions of use of this website.