Blood glucose is a vital indicator in the measurement of glucose metabolism. Blood glucose monitoring denotes the regular inspection of changes in human blood glucose levels, which is crucial for diabetic patients. Real-time monitoring can also significantly reduce the risk of diabetes complications, enhance blood glucose control, and improve the overall quality of life.
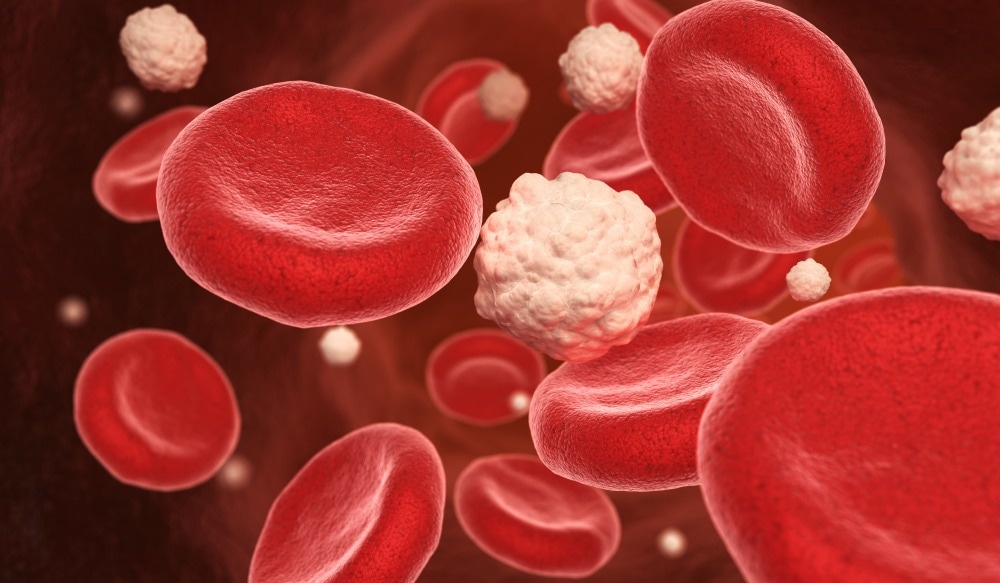
Image Credit: Tatiana Shepeleva/Shutterstock.com
Optical and electrochemical methods are the most common types of glucose detection methods used today. Optical detection methods are based on two main principles. The first is optical techniques without fluorescent chromophores, and the second is an implantable optical sensor that detects variations in glucose concentration using a fluorescent chromophore.
Because of its simplicity and adaptability colorimetric detection centered on the first principle has been widely used in point of care testing (POCT). Electrochemical detection is a method of detecting a target by transforming chemical signals into electrical signals.
Paper-based microfluidic sensors have taken up a large portion of the market for microfluidic sensors. Preparing paper-based sensors is time-consuming, and given the strong hydrophilicity of the paper base, they cannot be cleaned, and reagent loss will always occur during the flow.
In this study, the researchers propose a microfluidic sensor made of polyethylene terephthalate (PET). In comparison to paper-based sensors, the PET material has a certain toughness, which safeguards the electrodes from bending damage.
Methodology
All reagents used in the study were of analytical grade and were used without further purification, while all solutions were made with deionized water.
The structure of the glucose sensor is shown in Figure 1. Each sensor is made up of eight biosensing modules that measure the glucose level. Three different types of electrodes are included in each module.
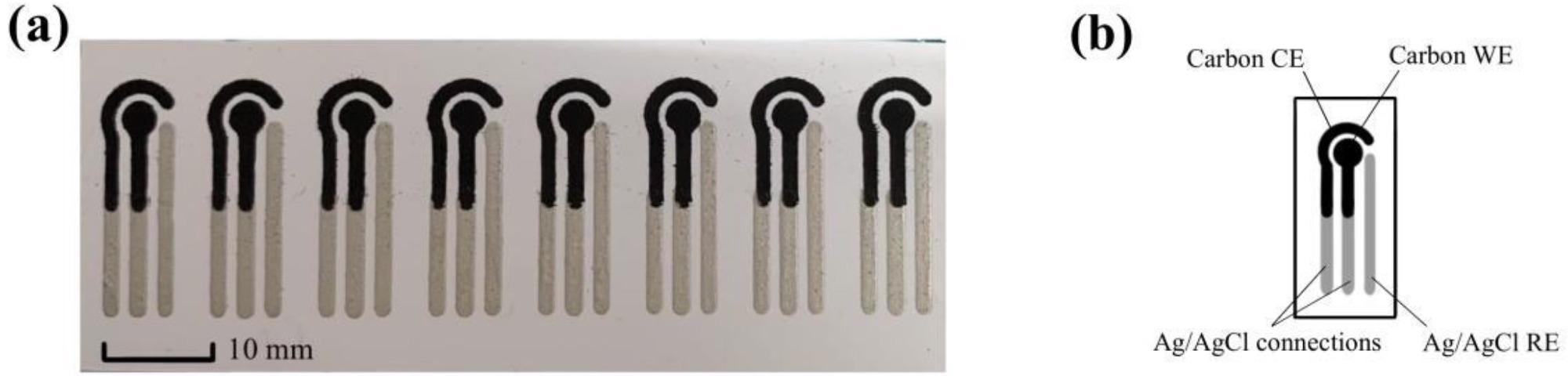
Figure 1. (a) Design of the glucose sensor. (b) Schematic illustration of a single module with three electrodes. Image Credit: Yang, et al., 2022
The researchers were able to cover the three electrodes uniformly with a very limited amount of sample and reagent owing to the natural hydrophobicity of the PET substrate, the presence of liquid surface tension, and the small size design of the electrodes. The electrodes made of Ag/AgCl ink were chosen because of their high electrical conductivity and strong adhesion to the PET substrate.
The working electrode was designed in the shape of a circle in terms of dimensions. An electrochemical sensor’s electrode is a critical component. Many methods for making electrodes have been proposed, with screen printing being one of the most popular due to its high efficiency and low cost. Figure 2 depicts the protocol of glucose sensor fabrication.
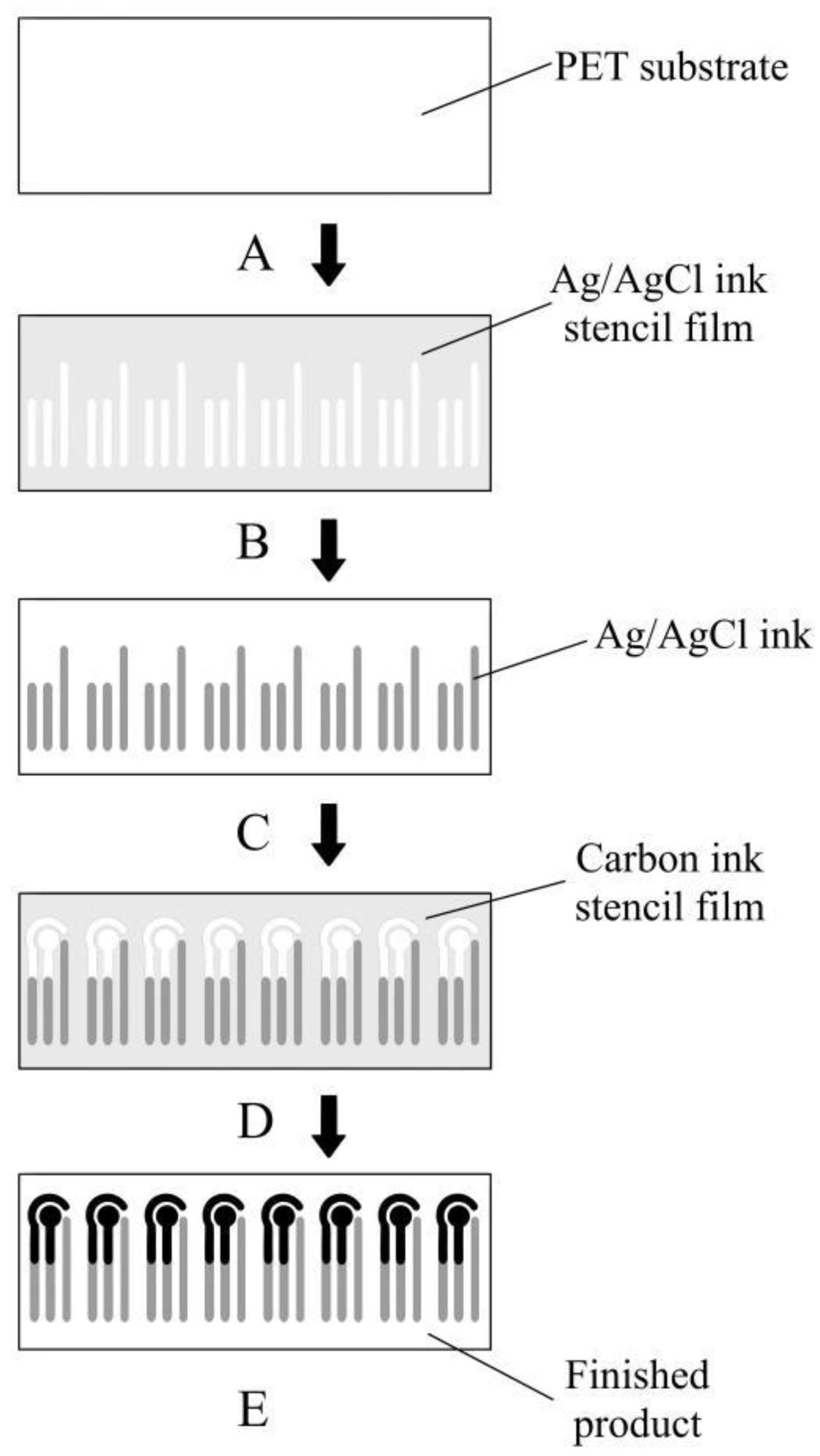
Figure 2. Fabrication process of the glucose sensor. Image Credit: Yang, et al., 2022
Glucose sensors are primarily based on enzymatic and non-enzymatic methods. Commercial sensors frequently employ enzymatic methods. Glucose oxidase and glucose dehydrogenase (GDH) are the most commonly used enzymes in commercial enzymatic sensors.
GOx is more widely used than GDH because it is less expensive, has better stability, and has a higher specificity for glucose. Potassium ferricyanide (K3[Fe(CN)6]) is a common oxidant used to more accurately estimate blood glucose levels.
The test zone was cleaned after the fabrication of the glucose sensor to eliminate potential contaminants that would have been introduced during the fabrication process. The reagents were configured before the experiment.
Results and Discussion
An electrochemical test platform, consisting of a commercial handheld potentiostat connection and a custom-made electrochemical glucose sensor was used to conduct all of the tests. One of the most significant techniques for electroanalytical chemistry research is cyclic voltammetry (CV).
The test results can also be used to characterize the sensor. To avoid reagent loss due to jitter during connection, the sensor was first connected to the electrochemical workstation. To observe the experimental results, CV waves with variable scan rates were applied sequentially.
The evaluated cyclic voltammograms disclosed typical reversible electrochemical reactions throughout all scan rates, as shown in Figure 3.
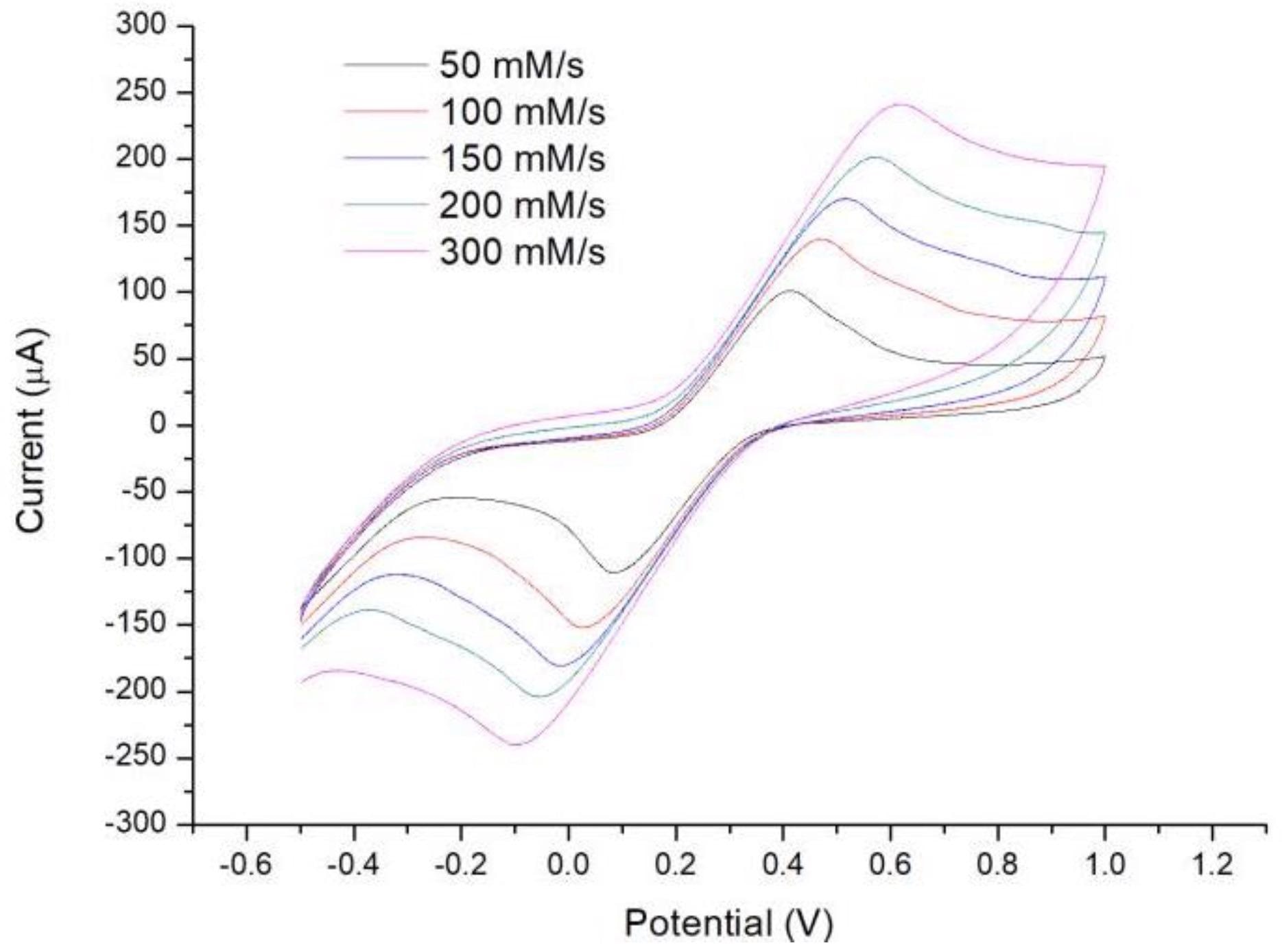
Figure 3. Measurement of cyclic voltammetry (CV) curves at scan rates of 50 mV/s, 100 mV/s, 150 mV/s, 200 mV/s, and 300 mV/s. Image Credit: Yang, et al., 2022
The square root of the scan rate was linearly proportional to the peak–peak current of the cyclic voltammogram (Figure 4), reiterating that the glucose sensor is a reversible electrochemical system.
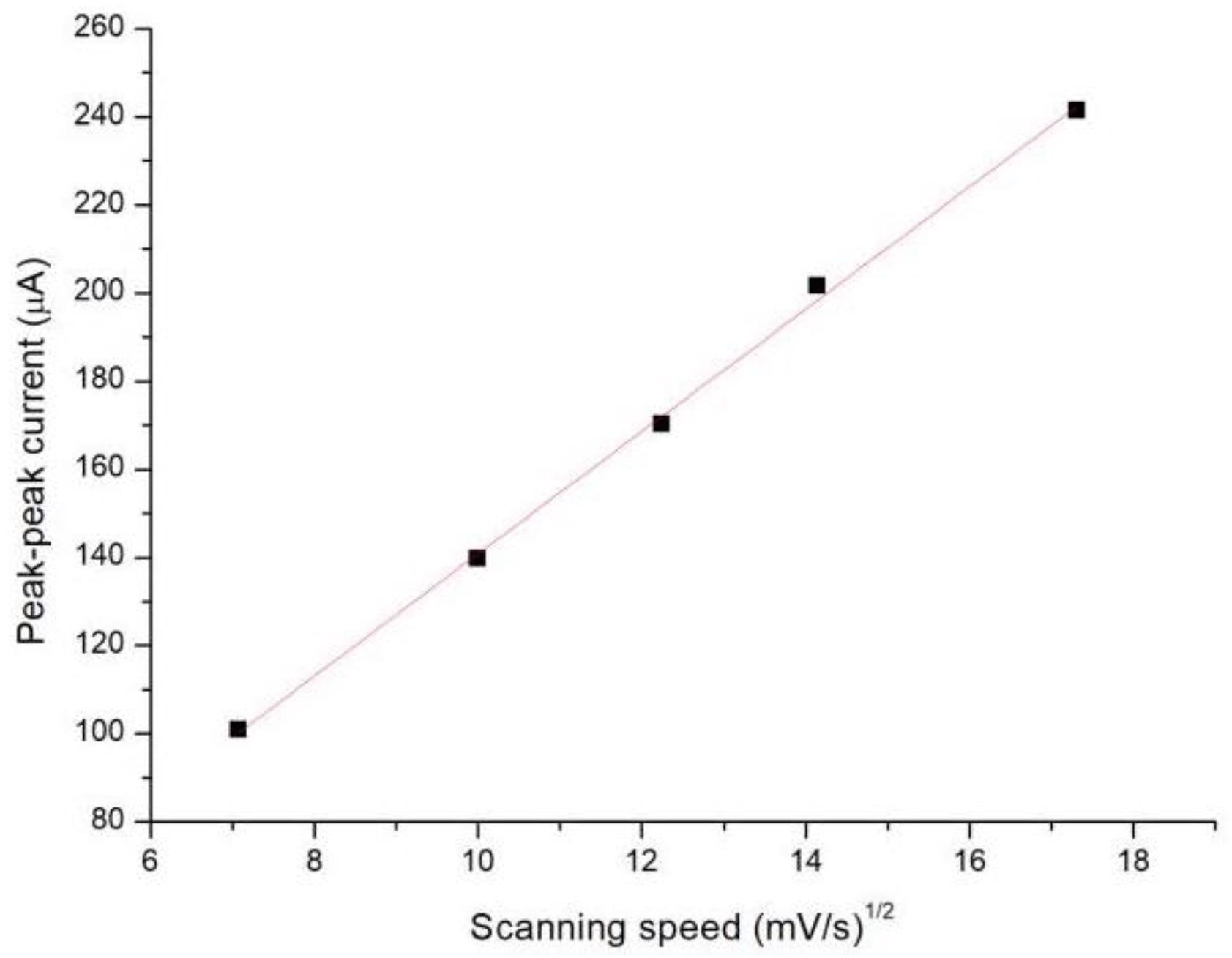
Figure 4. The peak–peak current of the CV curve versus the square root of the scan rate. Image Credit: Yang, et al., 2022
During fasting, human blood glucose levels typically range from 3.9 to 6.1 mM. In the blood glucose detection experiment, the ready-to-use sensor had to go through the same pre-processing as the CV measurement for each glucose test.
The sensor was first attached to the electrochemical workstation after preparation, and the solutions were placed in the test zone separately. Because chronoamperometry has better accuracy and sensitivity than the other electrochemical methods, it was preferred to quantify blood glucose levels. During the test, a 500 mV step potential was applied to the working electrode and evaluated the current versus time curve that resulted.
Simulated blood at 0, 5, 10, 15, 20, and 30 mM was used to examine the relationship between glucose concentration and steady-state Faraday current. The experimental results show that the current attained a steady-state within 120 seconds of applying the step potential, as depicted in Figure 5.
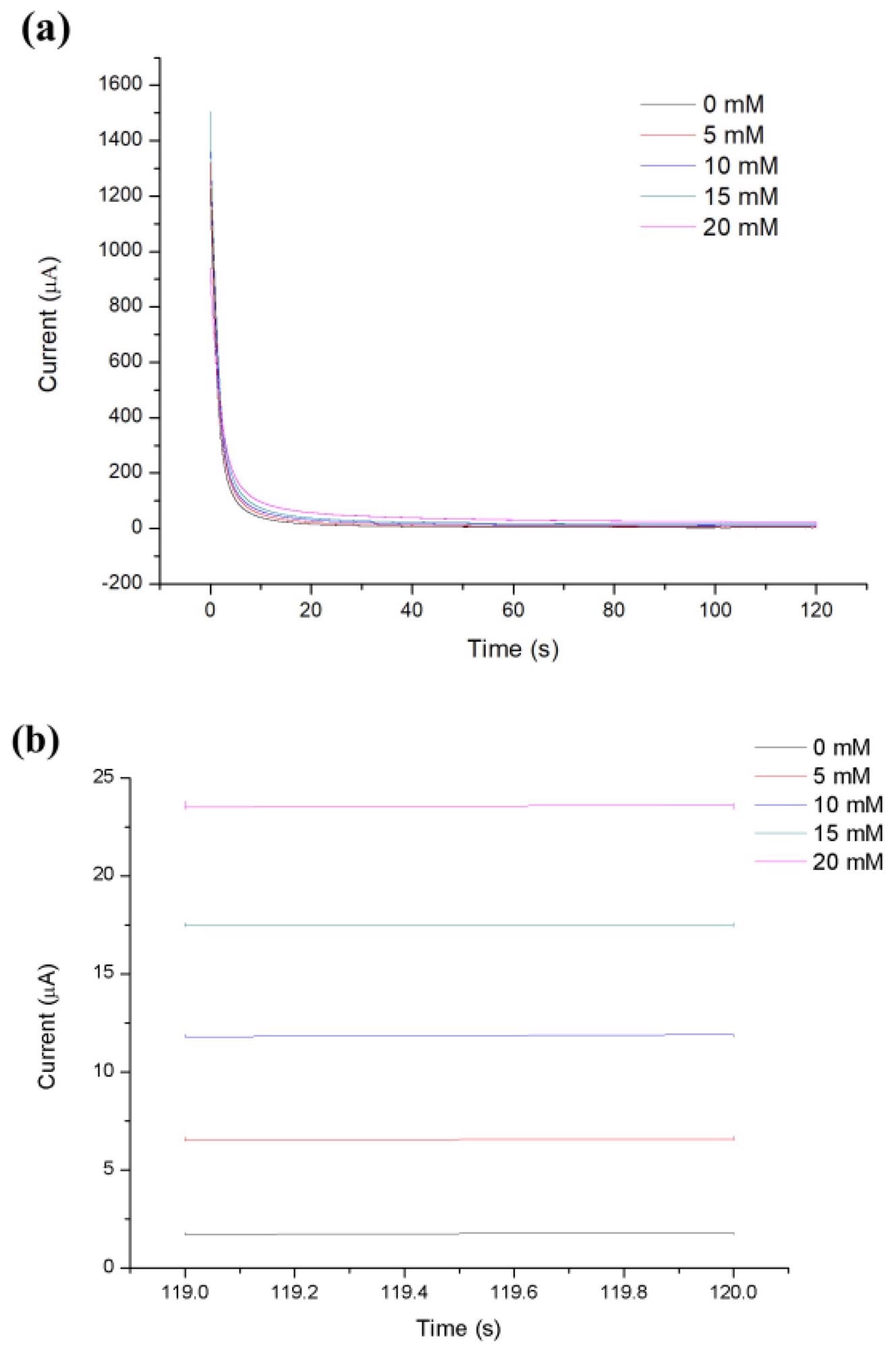
Figure 5. (a) Representative CA current curves measured at different concentrations. (b) The zoomed-in views of the CA current curves in (a) from 119 to 120s. Image Credit: Yang, et al., 2022
The Faradaic current evaluated was proportional to the glucose concentration, as explained by the Cottrell equation (Figure 6).
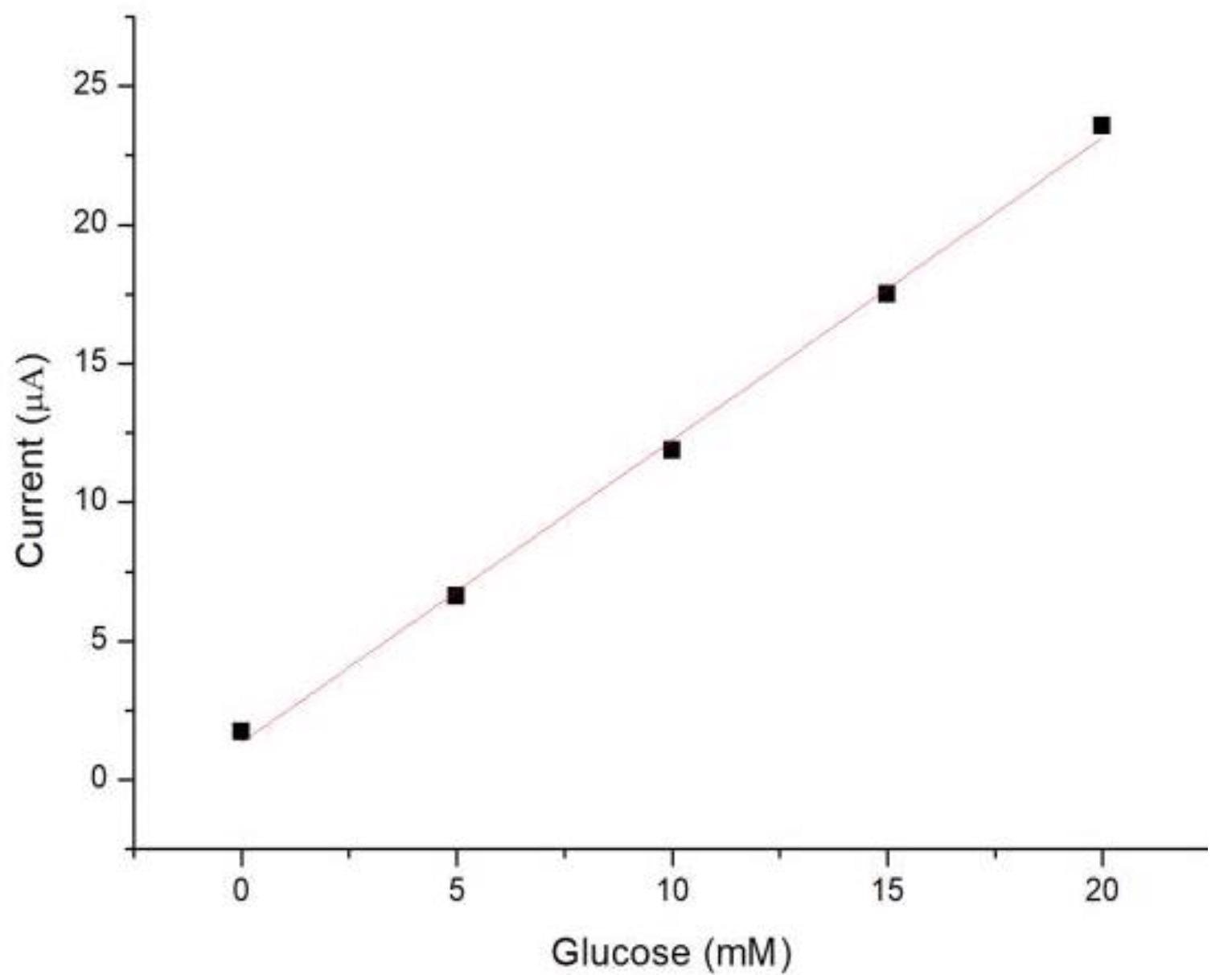
Figure 6. The calibration plot for the measurement of glucose. Image Credit: Yang, et al., 2022
The concentration that produced a current three times the standard deviation of currents assessed in zero-concentration simulated blood was calculated as the limit of detection (LOD). The glucose sensor’s LOD was 0.27 mM in this study, which is lower than that of a commercial blood glucose meter (0.83 mM).
Conclusion
In the current study, a PET-based microfluidic sensor was fabricated and tested with glucose as the target analyte. The fabricated sensor was electrochemically characterized using CV and could be used for biochemical analysis. The sensor is a reversible electrochemical system.
Enzyme-based electrochemical sensors were used to detect glucose. The steady-state Faraday current was found to be proportional to glucose concentration based on the experimental results, and a linear fit was performed based on the well-fitting experimental data. Eventually, the detection limit of this sensor was determined to be 0.23 mM, which is comparable to the detection limits of currently available commercial glucose meters.
Journal Reference:
Yang, L., Zhang, Z., Wang, X. (2022) A Microfluidic PET-Based Electrochemical Glucose Sensor. Micromachines, 13(4), p. 552. Available Online: https://www.mdpi.com/2072-666X/13/4/552/htm.
References and Further Reading
- Blonde, L & Karter, A J (2005) Current evidence regarding the value of self-monitored blood glucose testing. The American Journal of Medicine, 118, pp. 20–26. doi.org/10.1016/j.amjmed.2005.07.053.
- Zhang, L., et al. (2019) Portable glucose meter: Trends in techniques and its potential application in analysis. Analytical and Bioanalytical Chemistry, 411, p. 21. doi.org/10.1007/s00216-018-1361-7.
- Saeedi, P., et al. (2019) Global and regional diabetes prevalence estimates for 2019 and projections for 2030 and 2045: Results from the International Diabetes Federation Diabetes Atlas, 9th edition. Diabetes Research and Clinical Practice, 157, p. 107843. doi.org/10.1016/j.diabres.2019.107843.
- Gordon, C (2019) Blood glucose monitoring in diabetes: Rationale and procedure. The British Journal of Nursing, 28, pp. 434–439. doi.org/10.12968/bjon.2019.28.7.434.
- Tang, L., et al. (2020) Non-invasive blood glucose monitoring technology: A review. Sensors, 20, p. 6925. doi.org/10.3390/s20236925.
- Yang, X., et al. (2006) A fluorescent glucose biosensor based on immobilized glucose oxidase on bamboo inner shell membrane. Biosensors and Bioelectronics, 21, pp. 1613–1620. doi.org/10.1016/j.bios.2005.08.004.
- Martinez, A. W., et al. (2008) Simple telemedicine for developing regions: Camera phones and paper-based microfluidic devices for real-time, off-site diagnosis. Analytical Chemistry, 80, pp. 3699–3707. doi.org/10.1021/ac800112r.
- Dungchai, W., et al. (2010) Use of multiple colorimetric indicators for paper-based microfluidic devices. Analytica Chimica Acta, 674, pp. 227–233. doi.org/10.1016/j.aca.2010.06.019.
- Li, X., et al. (2013) Magnetic timing valves for fluid control in paper-based microfluidics. Lab Chip, 13, pp. 2609–2614. doi.org/10.1039/C3LC00006K.
- Zang, D., et al. (2013) A disposable simultaneous electrochemical sensor array based on a molecularly imprinted film at a NH2-graphene modified screen-printed electrode for determination of psychotropic drugs. Analyst, 138, pp. 2704–2711. doi.org/10.1039/C3AN00109A.
- Ge, S., et al. (2013) A disposable paper-based electrochemical sensor with an addressable electrode array for cancer screening. Chemical Communications, 48, pp. 9397–9399. doi.org/10.1039/C2CC34887J.
- Son, S. E., et al. (2019) Highly Sensitive Electrochemical Determination of Norepinephrine Using Poly Acrylic Acid-Coated Nanoceria. ChemElectroChem, 6, pp. 4666–4673. doi.org/10.1002/celc.201901222.
- Shervedani, R. K., et al. (2006) A novel method for glucose determination based on electrochemical impedance spectroscopy using glucose oxidase self-assembled biosensor. Bioelectrochemistry, 69, pp. 201–208. doi.org/10.1016/j.bioelechem.2006.01.003.
- Zhao, C & Liu, X (2016) A portable paper-based microfluidic platform for multiplexed electrochemical detection of human immunodeficiency virus and hepatitis C virus antibodies in serum. Biomicrofluidics, 10, p. 024119.
- Fu, H., et al. (2019) A paper-based microfluidic platform with shape-memory-polymer-actuated fluid valves for automated multi-step immunoassays. Microsystems & Nanoengineering, 5, p. 50. doi.org/10.1038/s41378-019-0091-0.
- Liao, S., et al. (2013) A capillary dielectrophoretic chip for real-time blood cell separation from a drop of whole blood. Biomicrofluidics, 7, p. 24110. doi.org/10.1063/1.4802269.
- Zaman, M. A., et al. (2021) Microparticle transport along a planar electrode array using moving dielectrophoresis. Journal of Applied Physics, 130, p. 034902. doi.org/10.1063/5.0049126.
- Eom, H., et al. (2022) Evaluating the electrochemical properties of supercapacitors using the three-electrode system. Journal of Visualized Experiments (styled JoVE), 179.
- Adkins, J., et al. (2015) Electrochemical paper-based microfluidic devices. Electrophoresis, 36, pp. 1811–1824. doi.org/10.1002/elps.201500084.
- Dawkins, R. C., et al. (2021) A screen-printed Ag/AgCl reference electrode with long-term stability for electroanalytical applications. Electrochimica Acta, 393, p. 139043. doi.org/10.1016/j.electacta.2021.139043.
- Sophocleous, M & Atkinson, J K (2017) A review of screen-printed silver/silver chloride (Ag/AgCl) reference electrodes potentially suitable for environmental potentiometric sensors. Sensors and Actuators A: Physical, 267, pp. 106–120. doi.org/10.1016/j.sna.2017.10.013.
- Hayat, A & Marty, J (2014) Disposable screen printed electrochemical sensors: Tools for environmental monitoring. Sensors, 14, pp. 10432–10453. doi.org/10.3390/s140610432.
- Søpstad, S., et al. (2018) Long-term stability of screen-printed pseudo-reference electrodes for electrochemical biosensors. Electrochimica Acta, 287, pp. 29–36. doi.org/10.1016/j.electacta.2018.08.045.
- Komoda, M., et al. (2019) Fabrication and characterization of a fully screen-printed Ag/AgCl reference electrode using silica gel inks exhibiting instantaneous usability and long-term stability. Electrochemistry, 87, pp. 65–69. doi.org/10.5796/electrochemistry.18-00075.
- Díaz-González, M., et al. (2005) Development of an immunosensor for the determination of rabbit IgG using streptavidin modified screen-printed carbon electrodes. Talanta, 65, pp. 565–573.
- Dungchai, W., et al. (2009) Electrochemical detection for paper-based microfluidics. Analytical Chemistry, 81, pp. 5821–5826. doi.org/10.1021/ac9007573.
- Zhao, C., et al. (2013) A microfluidic paper-based electrochemical biosensor array for multiplexed detection of metabolic biomarkers. Science and Technology of Advanced Materials, 14, p. 5. doi.org/10.1088/1468-6996/14/5/054402.
- Jangid, A.R., et al. (2019) Chronometric Quantitation of Analytes in Paper-Based Microfluidic Devices (MicroPADs) via Enzymatic Degradation of a Metastable Biomatrix. Inventions, 4, p. 48. doi.org/10.3390/inventions4030048.
- Shrivastava, A & Gupta, V (2011) Methods for the determination of limit of detection and limit of quantitation of the analytical methods. Chronicles of Young Scientists, 2, pp. 21–25. doi.org/10.4103/2229-5186.79345.
- Nie, Z., et al. (2010) Integration of paper-based microfluidic devices with commercial electrochemical readers. Lab Chip, 10, pp. 3163–3169. https://doi.org/10.1039/C0LC00237B.