Sensors for determining gases and volatile organic compounds (VOCs) have a wide range of applications. Most of these gas sensors contain semiconducting layers that change electrical resistance when exposed to gases and VOCs.
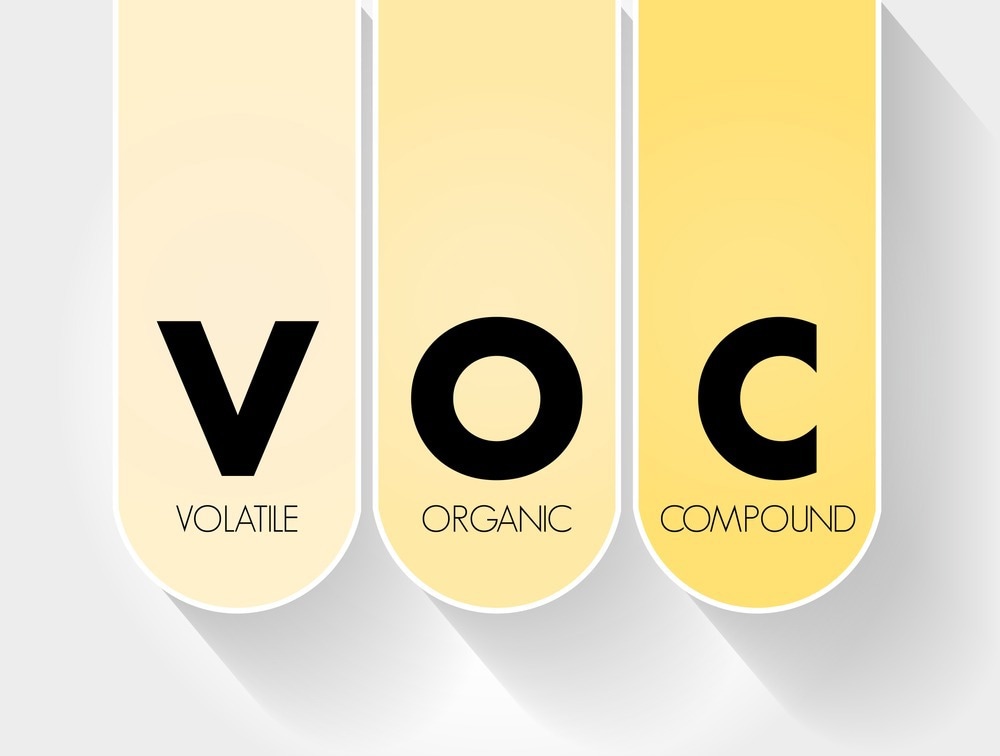
Image Credit: dizain/Shutterstock.com
These semiconducting layers are usually made of semiconductors like WO3, MoS2, ZnO, SnO2, and TiO2 TiOn, whereas the most popular dielectric substrates for gas sensors are Al2O3 and SiO2. New titanium-based compounds, such as MXenes, and non-stoichiometric titanium oxides (TiOn), have also been employed in gas sensor development.
The n-type stoichiometric TiO2 semiconductor, which exists in three primary phases (rutile, anatase, and brookite), may be easily changed between these phases using thermal techniques. Gas sensors can then be tuned analytically as a result.
In addition to stoichiometric TiO2 forms, a non-stoichiometric (TiOn) that is frequently referred to as TiO2-x has recently been used in sensor design. Structures of titanium oxide based on certain Magnéli phases (TinO2n-1) show great promise.
Some scientists have recently sought to improve gas-sensor selectivity by designing certain morphologies of semiconducting structures, using core-shell semiconducting nanocomposites, and quantum dots.
The characteristics, production, and modifications of TiO2-based nanostructures; use of titanium-based nanomaterials for energetics and environmental applications; the design of photo-catalysts; and the applicability of titanium oxides and MXenes in sensor design are all covered in recent studies. The progress in developing gas and volatile organic compound (VOC) sensors based on titanium-based oxides is the focus of a review published in the MDPI journal coatings.
Methodology
TiO2 is classified as an n-type semiconducting substance. Many sensors and biosensors are made with TiO2-based heterostructures. All of the most common types of titanium oxide, however, have distinct bandgaps, which are as follows: 3.02 eV for anatase, 3.23 eV for rutile, and 2.96 eV for brookite.
The addition of TiO2 (anatase) intergrowths to the structure of titanium pentoxide (Ti3O5) increases conductivity (Figure 1) as well as several photoluminescence-related properties.
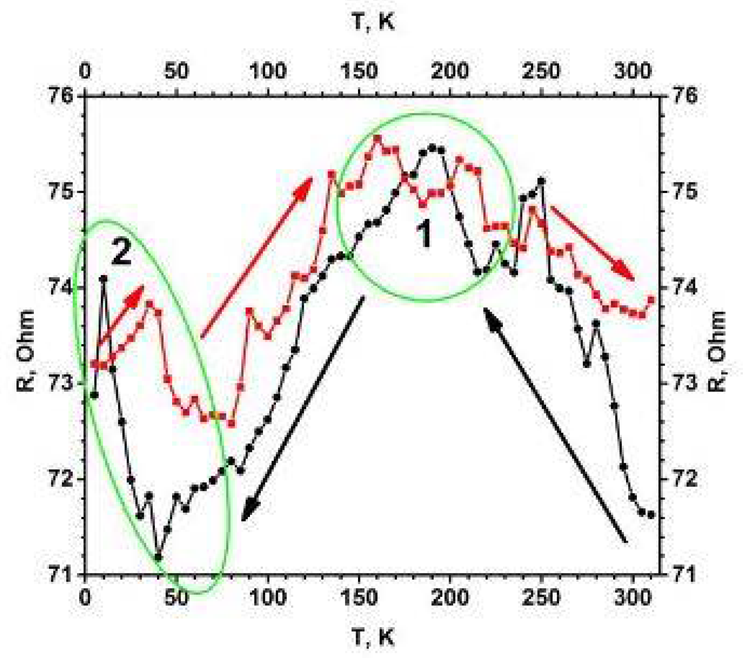
Figure 1. Temperature dependence of electrical resistance (R (T)) for the TiO2−x/TiO2 (400 °C)-based heterostructure. Temperature was changed in two ways (indicated by black and red arrows): (i) black cycles shows points measured by cooling down, (ii) red squares shows points by increasing temperature. Measurements were performed in vacuum using helium cryostat. Image Credit: Ramanavicius, et al., 2022
Gas sensors made on stoichiometric TiO2 exhibit remarkable sensitivity to several gases. It is worth noting that TiO2-based gas sensors can use a variety of detecting processes, which differ the most for determining reducing gaseous chemicals like H2, H2S, NH3, CO, CH3OH, C2H5OH, and others, as well as oxidizing gaseous compounds like O2, NO2, CO2 (Table 1).
Table 1. Characteristics of titanium oxide-based sensors. Source: Ramanavicius, et al., 2022
Sensing Material |
Working Temperature |
Gas Concentration |
Response Value (Ra/Rg) or ((ΔR/Rg) × 100%) |
Response Time |
Recovery Time |
Reference |
TiO2 (rutile), Ti8O15 and Ti9O17 mixture |
210 °C |
12.5–100 ppm (NH3) |
1–7% |
2 min |
8 min |
[68] |
TiOx-NiO |
250–350 °C |
100 ppm (H2)
100 ppm (NO2)
100 ppm (NH3) |
17 for H2 (250 °C)
16 for NO2 (250 °C)
4 for NH3 (250 °C) |
2 min |
2.3 min |
[69] |
β-Ti3O5 |
150 °C |
50 ppm (H2) |
11% |
- |
- |
[70] |
Ti3O5-TiO2 mixture |
25–180 °C |
105 ppm (H2O)
118 ppm (methanol)
53 ppm (ethanol)
18 ppm n-propanol
220 ppm (acetone) |
0.5–18% |
- |
4–35 s |
[1] |
TiO2-Ti6O |
150–450 °C |
2000 pm (H2)
20 ppm (NO2)
500 ppb (O3)
1.6 ppm (acetone)
80 ppm (NOx) |
2.9–348 |
8–21 s |
20–32 s |
[34] |
Ti3+-TiO2 |
RT |
100 ppm (CO) |
39% |
10 s |
30 s |
[71] |
TiO2 |
150 °C |
100 ppm (ethanol) |
75.4% |
155 s |
779 s |
[72] |
TiO2 |
270 °C |
500 ppm (acetone) |
9.19 |
10 s |
9 s |
[73] |
TiO2 |
350 °C |
400 ppm (ethanol) |
22.9 |
5 s |
7 s |
[74] |
TiO2 |
RT |
200 ppm (NH3) |
64 |
28 s |
24 s |
[75] |
α-Fe2O3-TiO2 |
325 °C |
100 ppm (ethanol) |
4 |
46 s |
16 s |
[76] |
UV irradiation enhances the activity of TiO2/GO-based heterostructures based on the generated n-n heterojunction, as seen in the band diagram in Figures 2a, b. The heterojunction bandgap in the TiO2/GO-based heterostructure is 4.7 eV, which is greater than the 4.4 eV seen in most GO-based structures. Figure 2b indicates that the produced heterostructure is composed of both accumulation and depletion layers.
UV irradiation can overcome this disadvantage by increasing the depletion layer in TiO2 and improving the accumulation layer in GO (Figure 2c). This lowered GO creates a p-n junction with TiO2, which has a narrower junction width at the contact (Figure 2d).
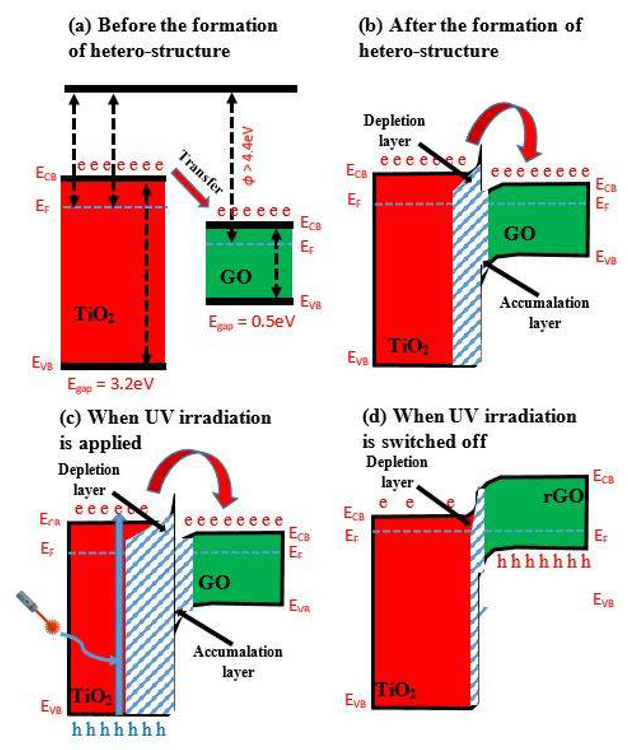
Figure 2. Band diagram of TiO2/GO heterostructure (a) before the formation of heterostructure, (b) after the formation of heterostructure, (c) when UV irradiation is applied, and (d) when UV irradiation is switched off. (‘e’ is an electron; ‘h’ is a hole). Image Credit: Ramanavicius, et al., 2022
Results
Both adsorption and desorption events, as well as the formation of new chemical bonds between the sensing TiO2 layer and adsorbed gas molecules, electrostatically affect the upper layer of the semiconducting TiO2 layer. The conductivity of this layer changes due to the depletion and enrichment of this layer by charge carriers.
Figure 3 depicts a typical analytical signal obtained by the adsorption and desorption of analyte gas.
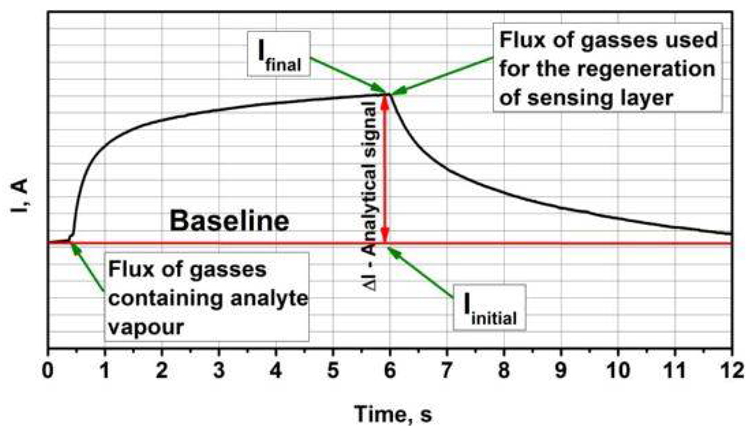
Figure 3. Representation of typical analytical signal for amperometric gas sensors. It should be noted that the duration of signal development and the regeneration of sensors highly depends on sensing material and sensing gases. Image Credit: Ramanavicius, et al., 2022
In TiO2-layers based on nano- or micro-particles, several distinct forms of electrical conductivity have been reported, including (i) Charge transmission via TiO2-particles is intrinsic, and (ii) charge transfer across the borders between particles is limited. As a result, the volume-concentration of these boundaries, as well as the particle size/shape, is crucial in constructing such sensors (Figure 4).
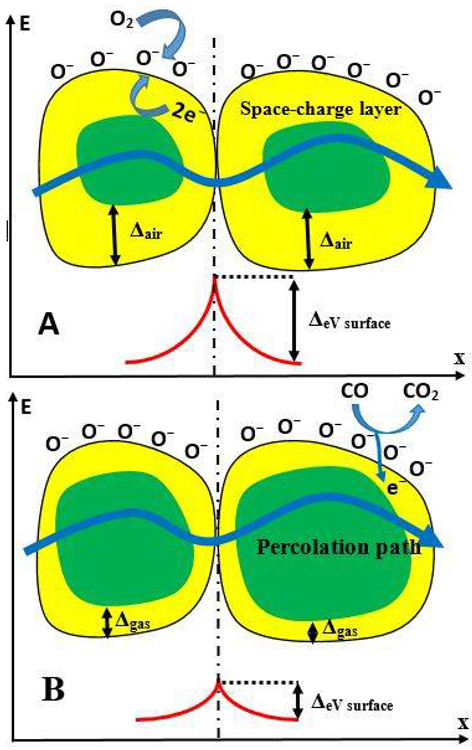
Figure 4. Representation of conductivity mechanism of semiconductor particle-based structure during the determination of CO gas. (A) Structure before the interaction with CO, (B) structure during the interaction with CO. Image Credit: Ramanavicius, et al., 2022
By combining metal oxide-based structures with conducting polymers, researchers could fine-tune various features of the produced composite material for specific purposes. As a result, several innovative techniques for synthesizing diverse morphologies TiO2 (Figure 5) and their composites with conducting polymers have been developed during the last decade.
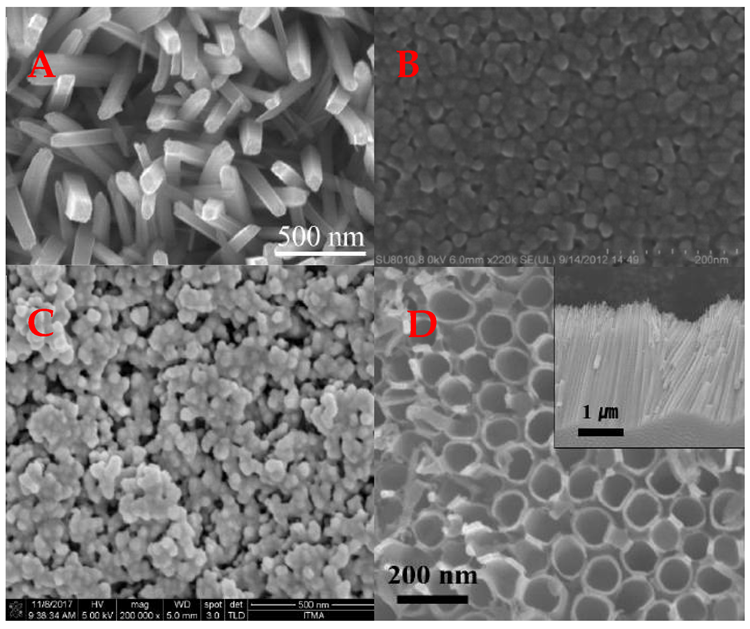
Figure 5. Different morphology TiO2 structures used in design of a gas sensors. (A) Nanorods, (B) thin film, (C) nanoparticles, (D) nanotubes. Image Credit: Ramanavicius, et al., 2022
Discussion
Most studies have shown that conducting the polymer polyaniline (PANI) via TiO2-based structures can boost their sensitivity to certain gases and VOCs. PANI modification of TiO2-based structures boosts the sensitivity of proposed ammonia sensors, according to a large number of studies.
Although all typical PANI forms (including leucoemeraldine and pernigraniline) may be employed to modify TiO2-based sensing layers, an emeraldine form of PANI appears to be the most appropriate. In addition to the most prevalent TiO2-forms (anatase and rutile), a new trend has emerged based on the use of nonstoichiometric titanium suboxides modified with conducting polymers such as PANI, polypyrrole (Ppy), polythiophene (PTH), and others.
Most (titanium oxide)/PANI-based sensors register analytical signals by measuring sensing layer resistance. There have been several publications on using quartz crystal microbalances (QCM) in the design of (titanium oxide)/PANI-based sensors. Trimethylamine, hydrazine, and NH3 were all detected using TiO2-PANI composite-based QCM sensors.
Conclusion
Gas sensors were designed using a variety of stoichiometric titanium oxide-based devices. However, selectivity issues persist in these sensors, which can be addressed by doping titanium oxide-based layers with dopants or forming heterostructures based on the combination of various semiconducting compounds, as well as adjusting the sensor’s working temperature.
Sensors made from composite materials based on titanium oxide modified with conducting polymers, such as PANI, are particularly promising, among many others.
Recent research has proven that TiO2/TiO2-x-based heterostructures may be successfully employed in the construction of gas sensors, with the detecting capabilities of these sensors being simply modified by modifying the TiO2/TiO2-x-ratio heterostructures.
Furthermore, TiO2/TiO2-x-based heterostructures are strong conductors, allowing them to operate in a “self-heating” mode and achieve temperatures adequate for determining certain gaseous chemicals.
The use of TiO2/TiO2-x-based heterostructures in the design of gas sensors is expected to become more common. Still, the main challenge in this research direction remains the control of stoichiometry and morphology of the TiO2/TiO2-x-based structure, which is critical for the sensitivity and selectivity of the designed gas sensors.
Journal Reference:
Ramanavicius, S., Arunas Jagminas, A. and Ramanavicius, A (2022) Gas Sensors Based on Titanium Oxides (Review). Coatings, 12(5), p.699. Available Online: https://www.mdpi.com/2079-6412/12/5/699/htm.
References and Further Reading
- Ramanavicius, S., et al. (2020) TiO2-x/TiO2-Structure Based ‘Self-Heated’ Sensor for the Determination of Some Reducing Gases. Sensors, 20(1), p. 74. doi.org/10.3390/s20010074.
- Ramanavicius, S & Ramanavicius, A (2020) Insights in the Application of Stoichiometric and Non-Stoichiometric Titanium Oxides for the Design of Sensors for the Determination of Gases and VOCs (TiO2−x and TinO2n−1 vs. TiO2). Sensors, 20(23), p. 6833. doi.org/10.3390/s20236833.
- Ramanavičius, S., et al. (2020) Selectivity of Tungsten Oxide Synthesized by Sol-Gel Method Towards Some Volatile Organic Compounds and Gaseous Materials in a Broad Range of Temperatures. Materials, 13(3), p. 523. doi.org/10.3390/ma13030523.
- Mirzaei, A., et al. (2018) Resistance-Based H2S Gas Sensors Using Metal Oxide Nanostructures: A Review of Recent Advances. Journal of Hazardous Materials, 357, pp. 314–331. doi.org/10.1016/j.jhazmat.2018.06.015.
- Mirzaei, A., et al. (2016) Detection of Hazardous Volatile Organic Compounds (VOCs) by Metal Oxide Nanostructures-Based Gas Sensors: A Review. Ceramics International, 42(14), pp. 15119–15141. doi.org/10.1016/j.ceramint.2016.06.145.
- Mirzaei, A., et al. (2018) How Shell Thickness Can Affect the Gas Sensing Properties of Nanostructured Materials: Survey of Literature. Sensors and Actuators B: Chemical, 258, pp. 270–294. doi.org/10.1016/j.snb.2017.11.066.
- Mirzaei, A., et al. (2018) Resistive-Based Gas Sensors for Detection of Benzene, Toluene and Xylene (BTX) Gases: A Review. Journal of Materials Chemistry C, 6(16), pp. 4342–4370. doi.org/10.1039/C8TC00245B.
- Petruleviciene, M., et al. (2020) Tuning the Photo-Luminescence Properties of WO3 Layers by the Adjustment of Layer Formation Conditions. Materials, 13(12), p. 2814. https://doi.org/10.3390/ma13122814
- Ramanavicius, S & Ramanavicius, A (2020) Progress and Insights in the Application of MXenes as New 2D Nano-Materials Suitable for Biosensors and Biofuel Cell Design. International Journal of Molecular Sciences, 21(23), p. 9224. doi.org/10.3390/ijms21239224.
- Wang, Y., et al. (2017) TiO2-Based Nanoheterostructures for Promoting Gas Sensitivity Performance: Designs, Developments, and Prospects. Sensors, 17(9), p. 1971. https://doi.org/10.3390/s17091971
- Bai, J & Zhou, B (2014) Titanium Dioxide Nanomaterials for Sensor Applications. Chemical Reviews, 114(19), pp. 10131–10176. doi.org/10.1021/cr400625j.
- Mokrushin, A. S., et al. (2021) Synthesis and Gas-Sensitive Chemoresistive Properties of TiO2:Cu Nanocomposite. Russian Journal of Inorganic Chemistry, 66, pp. 594–602. doi.org/10.1134/S0036023621040173.
- Mokrushin, A. S., et al. (2019) Oxygen Detection Using Nanostructured TiO2 Thin Films Obtained by the Molecular Layering Method. Applied Surface Science, 463, pp. 197–202. doi.org/10.1016/j.apsusc.2018.08.208.
- Sevastyanov, V. G., et al. (2018) Sol-Gel Made Titanium Dioxide Nanostructured Thin Films as Gas-Sensing Materials for the Detection of Oxygen. Mendeleev Communications, 28(2), pp. 164–166. doi.org/10.1016/j.mencom.2018.03.018.
- Mokrushin, A. S., et al. (2019) Gas-Sensing Properties of Nanostructured TiO2–XZrO2 Thin Films Obtained by the Sol–Gel Method. Journal of Sol-Gel Science and Technology, 92, pp. 415–426. doi.org/10.1007/s10971-019-04979-4.
- Simonenko, E. P., et al. (2019) A Sol-Gel Synthesis and Gas-Sensing Properties of Finely Dispersed ZrTiO4. Materials Chemistry and Physics, 225, pp. 347–357. doi.org/10.1016/j.matchemphys.2018.12.102.
- Simonenko, E. P., et al. (2019) Ink-Jet Printing of a TiO2–10%ZrO2 Thin Film for Oxygen Detection Using a Solution of Metal Alkoxoacetylacetonates. Thin Solid Films, 670, pp. 46–53. doi.org/10.1016/j.tsf.2018.12.004.
- Prades, J. D., et al. (2008) Ultralow Power Consumption Gas Sensors Based on Self-Heated Individual Nanowires. Applied Physics Letters, 93(12), p. 123110. doi.org/10.1063/1.2988265.
- Smulko, J. M., et al. (2015) New Approaches for Improving Selectivity and Sensitivity of Resistive Gas Sensors: A Review. Sensor Review, 35(4), pp. 340–347. doi.org/10.1108/SR-12-2014-0747.
- Simon, I., et al. (2001) Micromachined Metal Oxide Gas Sensors: Opportunities to Improve Sensor Performance. Sensors and Actuators B: Chemical, 73(1), pp. 1–26. doi.org/10.1016/S0925-4005(00)00639-0.
- Karnati, P., et al. (2019) Conduction Mechanisms in One Dimensional Core-Shell Nanostructures for Gas Sensing: A Review. Sensors and Actuators B: Chemical, 295, pp. 127–143. doi.org/10.1016/j.snb.2019.05.049.
- Kim, J. H., et al. (2018) Low Power-Consumption CO Gas Sensors Based on Au-Functionalized SnO2-ZnO Core-Shell Nanowires. Sensors and Actuators B: Chemical, 267, pp. 597–607. doi.org/10.1016/j.snb.2018.04.079.
- Mirzaei, A., et al. (2015) Synthesis, Characterization and Gas Sensing Properties of Ag@α-Fe2O3 Core–Shell Nanocomposites. Nanomaterials, 5(2), pp. 737–749. doi.org/10.3390/nano5020737.
- Deng, J., et al. (2016) Enhanced H2S Gas Sensing Properties of Undoped ZnO Nanocrystalline Films from QDs by Low-Temperature Processing. Sensors and Actuators B: Chemical, 224, pp. 153–158. doi.org/10.1016/j.snb.2015.10.022.
- Mosadegh Sedghi, S., et al. (2010) Low Temperature CO and CH4 Dual Selective Gas Sensor Using SnO2 Quantum Dots Prepared by Sonochemical Method. Sensors and Actuators B: Chemical, 145(1), pp. 7–12. doi.org/10.1016/j.snb.2009.11.002.
- Chen, X & Mao, S S (2007) Titanium Dioxide Nanomaterials: Synthesis, Properties, Modifications, and Applications. Chemical Reviews, 107(7), pp. 2891–2959. doi.org/10.1021/cr0500535.
- Zhang, Y., et al. (2015) Titanate and Titania Nanostructured Materials for Environmental and Energy Applications: A Review. RSC Advances, 5(97), pp. 79479–79510. doi.org/10.1039/C5RA11298B.
- Linsebigler, A. L., et al. (1995) Photocatalysis on TiO2 Surfaces: Principles, Mechanisms, and Selected Results. Chemical Reviews, 95(3), pp. 735–758. doi.org/10.1021/cr00035a013.
- Soni, P., et al. (2018) The Effect of Ni2+ Ions on Energy Band Gap of TiO2 Nanoparticles for Solar Cell Applications. Journal of Nanoscience, Nanoengineering & Applications, 8(2), pp. 69–74.
- Yamazoe, N., et al. (2003) Oxide Semiconductor Gas Sensors. Catalysis Surveys from Asia, 7, pp. 63–75. doi.org/10.1023/A:1023436725457.
- Wang, G., et al. (2016) Anodization Fabrication of 3D TiO2 Photonic Crystals and Their Application for Chemical Sensors. Superlattices and Microstructures, 100, pp. 290–295. doi.org/10.1016/j.spmi.2016.09.027.
- Si, H., et al. (2019) A Real-Time on-Line Photoelectrochemical Sensor toward Chemical Oxygen Demand Determination Based on Field-Effect Transistor Using an Extended Gate with 3D TiO2 Nanotube Arrays. Sensors and Actuators B: Chemical, 289, pp. 106–113. doi.org/10.1016/j.snb.2019.03.071.
- Qiu, J., et al. (2011) Recent Applications of TiO2 Nanomaterials in Chemical Sensing in Aqueous Media. Sensors and Actuators B: Chemical, 160(1), pp. 875–890. doi.org/10.1016/j.snb.2011.08.077.
- Maziarz, W., et al. (2016) Nanostructured TiO2-Based Gas Sensors with Enhanced Sensitivity to Reducing Gases. Beilstein Journal of Nanotechnology, 7, pp. 1718–1726. doi.org/10.3762/bjnano.7.164.
- Tereshchenko, A., et al. (2018) Interaction Mechanism between TiO2 Nanostructures and Bovine Leukemia Virus Proteins in Photoluminescence-Based Immunosensors. RSC Advances, 8(66), pp. 37740–37748. doi.org/10.1039/C8RA07347C.
- Tereshchenko, A., et al. (2013) TiO2 Optical Sensor for Amino Acid Detection. In: Biophotonics—Riga 2013, Riga, Latvia, 26–31 August 2013; Spigulis, J., Kuzmina, I., Eds.; SPIE: Washington, DC, USA, 2013; Volume 9032, pp. 186–190. https://doi.org/10.1117/12.2044464.
- Wunderlich, W., et al. (2004) ELECTRONIC PROPERTIES OF NANO-POROUS TiO 2- AND ZnO THIN FILMS- COMPARISON OF SIMULATIONS AND EXPERIMENTS. Journal of Ceramic Processing Research, 5(4), pp. 343–354.
- Åsbrink, S & Magnéli, A (1959) Crystal Structure Studies on Trititanium Pentoxide, Ti3O5. Acta Crystallographica, 12, pp. 575–581. doi.org/10.1107/S0365110X59001694.
- Hong, S -H & Åsbrink, S (1982) The Structure of γ-Ti3O5 at 297 K. Acta Crystallographica Section B, 38, pp. 2570–2576. doi.org/10.1107/S056774088200939X.
- Onoda, M (1998) Phase Transitions of Ti3O5. Journal of Solid State Chemistry, 136(1), pp. 67–73. doi.org/10.1006/jssc.1997.7657.
- Ohkoshi, S., et al. (2010) Synthesis of a Metal Oxide with a Room-Temperature Photoreversible Phase Transition. Nature Chemistry, 2, pp. 539–545. doi.org/10.1038/nchem.670.
- Tanaka, K., et al. (2015) Structural Phase Transition between γ-Ti3O5 and δ-Ti3O5 by Breaking of a One-Dimensionally Conducting Pathway. Crystal Growth & Design, 15(2), 653–657. doi.org/10.1021/cg5013439.
- Yoshimatsu, K., et al. (2017) Superconductivity in Ti4O7 and γ-Ti3O5 Films. Scientific Reports, 7, p. 12544. doi.org/10.1038/s41598-017-12815-4.
- Marezio, M., et al. (1973) Structural Aspects of the Metal-Insulator Transitions in Ti4O7. Journal of Solid State Chemistry, 6(2), pp. 213–221. doi.org/10.1016/0022-4596(73)90184-9.
- Lakkis, S., et al. (1976) Metal-Insulator Transitions in Ti4O7 Single Crystals: Crystal Characterization, Specific Heat, and Electron Paramagnetic Resonance. Physical Review B, 14(4), pp. 1429–1440. doi.org/10.1103/PhysRevB.14.1429.
- D’Angelo, A M & Webster, N A S (2018) Evidence of Anatase Intergrowths Formed during Slow Cooling of Reduced Ilmenite. Journal of Applied Crystallography, 51, pp. 185–192. doi.org/10.1107/S1600576718000493.
- Grey, I. E., et al. (2000) New M3O5-Anatase Intergrowth Structures Formed during Low-Temperature Oxidation of Anosovite. Journal of Solid State Chemistry, 150(1), pp. 128–138. doi.org/10.1006/jssc.1999.8564.
- Jayashree, S & Ashokkumar, M (2018) Switchable Intrinsic Defect Chemistry of Titania for Catalytic Applications. Catalysts, 8(12), 601. doi.org/10.3390/catal8120601.
- Andersson, S & Magnéli, A (1956) Diskrete Titanoxydphasen Im Zusammensetzungsbereich TiO_1,75-TiO_1,90. Naturwissenschaften, 43, pp. 495–496. doi.org/10.1007/BF00632520.
- Liborio, L., et al. (2009) Electronic Structure of the Ti4O7 Magneli Phase. Physical Review B, 79(24), p. 245133. doi.org/10.1103/PhysRevB.79.245133.
- Liborio, L & Harrison, N (2008) Thermodynamics of Oxygen Defective Magn\’eli Phases in Rutile: A First-Principles Study. Physical Review B, 77(10), p. 104104. doi.org/10.1103/PhysRevB.77.104104.
- Adamaki, V., et al. (2014) Manufacturing and Characterization of Magnéli Phase Conductive Fibres. Journal of Materials Chemistry A, 2(22), pp. 8328–8333. doi.org/10.1039/C4TA00685B.
- Zhu, Q., et al. (2014) Stable Blue TiO2−x Nanoparticles for Efficient Visible Light Photocatalysts. Journal of Materials Chemistry A, 2(12), pp. 4429–4437. doi.org/10.1039/C3TA14484D.
- Al-Hashem, M., et al. (2019) Role of Oxygen Vacancies in Nanostructured Metal-Oxide Gas Sensors: A Review. Sensors and Actuators B: Chemical, 301, p. 126845. doi.org/10.1016/j.snb.2019.126845.
- Seebauer, E G & Kratzer, M C (2006) Charged Point Defects in Semiconductors. Materials Science and Engineering: R: Reports, 55(3–6), pp. 57–149. doi.org/10.1016/j.mser.2006.01.002.
- Harada, S., et al. (2010) Thermoelectric Properties and Crystallographic Shear Structures in Titanium Oxides of the Magnèli Phases Bandgap Engineering of Magnéli Phase TinO2n−1: Electron-Hole Self-Compensation. Journal of Applied Physics, 108(8), p. 83703. doi.org/10.1063/1.3498801.
- Smith, J. R., et al. (1998) Electrodes Based on Magnéli Phase Titanium Oxides: The Properties and Applications of Ebonex® Materials. Journal of Applied Electrochemistry, 28, pp. 1021–1033. doi.org/10.1023/A:1003469427858
- Walsh, F C & Wills, R G A (2010) The Continuing Development of Magnéli Phase Titanium Sub-Oxides and Ebonex® Electrodes. Electrochimica Acta, 55(22), pp. 6342–6351. doi.org/10.1016/j.electacta.2010.05.011.
- Nakamura, I., et al. (2000) Role of Oxygen Vacancy in the Plasma-Treated TiO2 Photocatalyst with Visible Light Activity for NO Removal. Journal of Molecular Catalysis A: Chemical, 161(1–2), pp. 205–212. doi.org/10.1016/S1381-1169(00)00362-9.
- le Mercier, T., et al. (1995) Formation of Ti3+ Ions at the Surface of Laser-Irradiated Rutile. Applied Surface Science, 86(1–4), pp. 382–386. doi.org/10.1016/0169-4332(94)00421-8.
- Zheng, Z., et al. (2013) Metallic Zinc- Assisted Synthesis of Ti3+ Self-Doped TiO2 with Tunable Phase Composition and Visible-Light Photocatalytic Activity. Chemical Communications, 49(9), pp. 868–870. doi.org/10.1039/C2CC37976G.
- Hashimoto, S & Tanaka, A (2002) Alteration of Ti 2p XPS Spectrum for Titanium Oxide by Low-Energy Ar Ion Bombardment. Surface and Interface Analysis, 34(1), pp. 262–265. doi.org/10.1002/sia.1296.
- Wang, Y., et al. (2008) Nanostructured Sheets of Ti—O Nanobelts for Gas Sensing and Antibacterial Applications. Advanced Functional Maerials, 18(7), pp. 1131–1137. doi.org/10.1002/adfm.200701120.
- Hayfield, P C S (2007) Development of a New Material: Monolithic Ti4O7 Ebonex Ceramic; In: Royal Society of Chemistry: London, UK, 2007; ISBN 184755069X.
- Kimura, M., et al. (2012) Sensing of Vaporous Organic Compounds by TiO2 Porous Films Covered with Polythiophene Layers. Advanced Functional Maerials, 22(3), pp. 469–476. doi.org/10.1002/adfm.201101953.
- Viter, R., et al. (2017) Toward Development of Optical Biosensors Based on Photoluminescence of TiO2 Nanoparticles for the Detection of Salmonella. Sensors and Actuators B: Chemical, 252, pp. 95–102. doi.org/10.1016/j.snb.2017.05.139.
- Haryński, Ł., et al. (2020) Scalable Route toward Superior Photoresponse of UV-Laser-Treated TiO2 Nanotubes. ACS Applied Materials & Interfaces, 12(2), pp. 3225–3235. doi.org/10.1021/acsami.9b19206.
- Gardon, M., et al. (2013) New Procedures for Building-up the Active Layer of Gas Sensors on Flexible Polymers. Surface and Coatings Technology, 235, pp. 848–852. doi.org/10.1016/j.surfcoat.2013.09.011.
- Imawan, C., et al. (2000) TiOx-Modified NiO Thin Films for H2 Gas Sensors: Effects of TiOx-Overlayer Sputtering Parameters. Sensors and Actuators B: Chemical, 68(1–3), pp. 184–188. doi.org/10.1016/S0925-4005(00)00427-5.
- Li, X., et al. (2015) The Synthesis and Gas Sensitivity of the β-Ti3O5 Powder: Experimental and DFT Study. Journal of Alloys and Compounds, 649, pp. 939–948. doi.org/10.1016/j.jallcom.2015.07.094.
- Su, J., et al. (2013) Porous Titania with Heavily Self-Doped Ti3+ for Specific Sensing of CO at Room Temperature. Inorganic Chemistry, 52(10), pp. 5924–5930. doi.org/10.1021/ic400109j.
- Gakhar, T & Hazra, A (2020) Oxygen Vacancy Modulation of Titania Nanotubes by Cathodic Polarization and Chemical Reduction Routes for Efficient Detection of Volatile Organic Compounds. Nanoscale, 12(16), pp. 9082–9093. doi.org/10.1039/C9NR10795A.
- Navale, S. T., et al. (2018) Enhanced Acetone Sensing Properties of Titanium Dioxide Nanoparticles with a Sub-Ppm Detection Limit. Sensors and Actuators B: Chemical, 255(P-2), pp. 1701–1710. doi.org/10.1016/j.snb.2017.08.186.
- Gao, X., et al. (2017) Hydrothermal Synthesis of Agglomerating TiO2 Nanoflowers and Its Gas Sensing. Journal of Materials Science: Materials in Electronics, 28, pp. 18781–18786. doi.org/10.1007/s10854-017-7827-0.
- Mintcheva, N., et al. (2020) Room-Temperature Gas Sensing of Laser-Modified Anatase TiO2 Decorated with Au Nanoparticles. Applied Surface Science, 507, p. 145169. doi.org/10.1016/j.apsusc.2019.145169.
- Mei, H., et al. (2020) Construction of Pine-Branch-like α-Fe2O3/TiO2 Hierarchical Heterostructure for Gas Sensing. Ceramics International, 46(11 P-B), pp. 18675–18682. doi.org/10.1016/j.ceramint.2020.04.181.
- Hsu, K. C., et al. (2019) Response and Characteristics of TiO2/Perovskite Heterojunctions for CO Gas Sensors. Journal of Alloys and Compounds, 794, pp. 576–584. doi.org/10.1016/j.jallcom.2019.04.238.
- Avansi, W., et al. (2019) One-Dimensional V2O5/TiO2 Heterostructures for Chemiresistive Ozone Sensors. ACS Applied Nano Materials, 2(8), pp. 4756–4764. doi.org/10.1021/acsanm.9b00578
- Chen, K., et al. (2019) SnO2 Nanoparticles/TiO2 Nanofibers Heterostructures: In Situ Fabrication and Enhanced Gas Sensing Performance. Solid-State Electronics, 157, pp. 42–47. doi.org/10.1016/j.sse.2019.03.024
- Yu, Q., et al. (2015) Facile Synthesis of α-Fe2O3@SnO2 Core–Shell Heterostructure Nanotubes for High Performance Gas Sensors. Sensors and Actuators B: Chemical, 213, pp. 27–34. doi.org/10.1016/j.snb.2015.01.130.
- Seekaew, Y., et al. (2019) Room Temperature Toluene Gas Sensor Based on TiO2 Nanoparticles Decorated 3D Graphene-Carbon Nanotube Nanostructures. Sensors and Actuators B: Chemical, 279, pp. 69–78. doi.org/10.1016/j.snb.2018.09.095.
- Lee, E., et al. (2018) Enhanced Gas-Sensing Performance of GO/TiO2 Composite by Photocatalysis. Sensors, 18(10), p. 3334. doi.org/10.3390/s18103334.
- Stratakis, E., et al. (2014) Improving the Efficiency of Organic Photovoltaics by Tuning the Work Function of Graphene Oxide Hole Transporting Layers. Nanoscale, 6(12), pp. 6925–6931. doi.org/10.1039/c4nr01539h.
- Chen, C., et al. (2010) Synthesis of Visible-Light Responsive Graphene Oxide/TiO2 Composites with p/n Heterojunction. ACS Nano, 4(11), pp. 6425–6432. doi.org/10.1021/nn102130m.
- Lightcap, I. V., et al. (2010) Anchoring Semiconductor and Metal Nanoparticles on a Two-Dimensional Catalyst Mat. Storing and Shuttling Electrons with Reduced Graphene Oxide. Nano Letters, 10(2), pp. 577–583. doi.org/10.1021/nl9035109.
- Ammu, S., et al. (2012) Flexible, All-Organic Chemiresistor for Detecting Chemically Aggressive Vapors. Journal of the American Chemical Society, 134(10), pp. 4553–4556. doi.org/10.1021/ja300420t.
- Lam, K. C., et al. (2017) Room-Temperature Methane Gas Sensing Properties Based on in Situ Reduced Graphene Oxide Incorporated with Tin Dioxide. Journal of Materials Chemistry A, 5(22), pp. 11131–11142. doi.org/10.1039/C7TA01293D.
- Ye, Z., et al. (2016) Room Temperature Formaldehyde Sensor with Enhanced Performance Based on Reduced Graphene Oxide/Titanium Dioxide. Sensors and Actuators B: Chemical, 223, pp. 149–156. doi.org/10.1016/j.snb.2015.09.102.
- Buchsteiner, A., et al. (2006) Water Dynamics in Graphite Oxide Investigated with Neutron Scattering. The Journal of Physical Chemistry B, 110(45), pp. 22328–22338. doi.org/10.1021/jp0641132.
- Phan, D T & Chung, G S (2015) Effects of Rapid Thermal Annealing on Humidity Sensor Based on Graphene Oxide Thin Films. Sensors and Actuators B: Chemical, 220, pp. 1050–1055. doi.org/10.1016/j.snb.2015.06.055.
- Wang, P., et al. (2011) Synthesis of reduced grapheneoxide-anatase TiO2 nanocomposite and its improved photo-induced charge transfer properties. Nanoscale, 3(4), pp. 1640–1645. doi.org/10.1039/C0NR00714E.
- Cui, S., et al. (2015) Stabilizing MoS2 Nanosheets through SnO2 Nanocrystal Decoration for High-Performance Gas Sensing in Air. Nano-Micro Small, 11(19), pp. 2305–2313. doi.org/10.1002/smll.201402923.
- Mirzaei, A., et al. (2015) Metal-Core@metal Oxide-Shell Nanomaterials for Gas-Sensing Applications: A Review. Journal of Nanoparticle Research, 17, 371. doi.org/10.1007/s11051-015-3164-5.
- Rieu, M., et al. (2016) Fully Inkjet Printed SnO2 Gas Sensor on Plastic Substrate. Sensors and Actuators B: Chemical, 236, pp. 1091–1097. doi.org/10.1016/j.snb.2016.06.042.
- Chung, F. C., et al. (2014) Fabrication of a Au@SnO2 Core–Shell Structure for Gaseous Formaldehyde Sensing at Room Temperature. Sensors and Actuators B: Chemical, 190, pp. 1–7. doi.org/10.1016/j.snb.2013.08.037
- Chen, G., et al. (2015) High-Energy Faceted SnO2-Coated TiO2 Nanobelt Heterostructure for Near-Ambient Temperature-Responsive Ethanol Sensor. ACS Applied Materials & Interfaces, 7(44), pp. 24950–24956. doi.org/10.1021/acsami.5b08630.
- Li, F., et al. (2017) Study on TiO2-SnO2 Core-Shell Heterostructure Nanofibers with Different Work Function and Its Application in Gas Sensor. Sensors and Actuators B: Chemical, 248, pp. 812–819. doi.org/10.1016/j.snb.2016.12.009.
- Zeng, W., et al. (2010) UV Light Activation of TiO2 Doped SnO2 Thick Film for Sensing Ethanol at Room Temperature. Materials Transactions, 51(2), pp. 243–245. doi.org/10.2320/matertrans.MC200904.
- Lee, H C & Hwang, W S (2006) Substrate Effects on the Oxygen Gas Sensing Properties of SnO2/TiO2 Thin Films. Applied Surface Science, 253(4), pp. 1889–1897. doi.org/10.1016/j.apsusc.2006.03.036.
- Lee, J. H., et al. (2020) Gas-Sensing Behaviors of TiO2-Layer-Modified SnO2 Quantum Dots in Self-Heating Mode and Effects of the TiO2 Layer. Sensors and Actuators B: Chemical, 310, p. 127870. doi.org/10.1016/j.snb.2020.127870.
- Ng, S., et al. (2020) Atomic Layer Deposition of SnO2-Coated Anodic One-Dimensional TiO2 Nanotube Layers for Low Concentration NO2 Sensing. ACS Applied Materials & Interfaces, 12(29), 33386–33396. doi.org/10.1021/acsami.0c07791.
- Song, Z., et al. (2016) Sensitive Room-Temperature H2S Gas Sensors Employing SnO2 Quantum Wire/Reduced Graphene Oxide Nanocomposites. Chemistry of Materials, 28, pp. 1205–1212. doi.org/10.1021/acs.chemmater.5b04850
- Nasriddinov, A., et al. (2019) Sub-Ppm Formaldehyde Detection by n-n TiO2@SnO2 Nanocomposites. Sensors, 19(14), p. 3182. doi.org/10.3390/s19143182.
- Li, X., et al. (2015) Highly Sensitive and Selective Room-Temperature Formaldehyde Sensors Using Hollow TiO2 Microspheres. Sensors and Actuators B: Chemical, 219, pp. 158–163. doi.org/10.1016/j.snb.2015.05.031.
- Righettoni, M., et al. (2009) Minimal Cross-Sensitivity to Humidity during Ethanol Detection by SnO2-TiO2 Solid Solutions Related Content Toward Portable Breath Acetone Analysis for Diabetes Detection Minimal Cross-Sensitivity to Humidity during Ethanol Detection by SnO2-TiO2 Solid Solutions. Nanotechnology, 20(31), p. 315502. doi.org/10.1088/0957-4484/20/31/315502.
- Li, Z., et al. (2018) Resistive-Type Hydrogen Gas Sensor Based on TiO2: A Review. International Journal of Hydrogen Energy, 43, pp. 21114–21132. doi.org/10.1016/j.ijhydene.2018.09.051.
- Shaposhnik, D., et al. (2012) Hydrogen Sensors on the Basis of SnO2–TiO2 Systems. Sensors and Actuators B: Chemical, 174, pp. 527–534. doi.org/10.1016/j.snb.2012.05.028.
- Plecenik, T., et al. (2015) Fast Highly-Sensitive Room-Temperature Semiconductor Gas Sensor Based on the Nanoscale Pt–TiO2–Pt Sandwich. Sensors and Actuators B: Chemical, 207, pp. 351–361. doi.org/10.1016/j.snb.2014.10.003.
- Lin, J., et al. (2014) 3D Hierarchical Rutile TiO2 and Metal-Free Organic Sensitizer Producing Dye-Sensitized Solar Cells 8.6% Conversion Efficiency. Scientific Reports, 4, p. 5769. doi.org/10.1038/srep05769
- Wang, C., et al. (2010) Metal Oxide Gas Sensors: Sensitivity and Influencing Factors. Sensors, 10(3), pp. 2088–2106. doi.org/10.3390/s100302088.
- Franke, M. E., et al. (2006) Metal and Metal Oxide Nanoparticles in Chemiresistors: Does the Nanoscale Matter? Nano-Micro Small, 2(1), pp. 36–50. doi.org/10.1002/smll.200500261.
- Wang, C., et al. (2010) Large Scale Synthesis and Gas-Sensing Properties of Anatase TiO2 Three-Dimensional Hierarchical Nanostructures. Langmuir, 26(15), pp. 12841–12848. doi.org/10.1021/la100910u
- Barreca, D., et al. (2007) First Example of ZnO−TiO2 Nanocomposites by Chemical Vapor Deposition: Structure, Morphology, Composition, and Gas Sensing Performances. Chemistry of Materials, 19(23), pp. 5642–5649. doi.org/10.1021/cm701990f.
- Lü, R., et al. (2013) Alumina Decorated TiO2 Nanotubes with Ordered Mesoporous Walls as High Sensitivity NOx Gas Sensors at Room Temperature. Nanoscale, 5(18), pp. 8569–8576. doi.org/10.1039/C3NR01903A
- Li, Z., et al. (2014) Ni-Doped TiO2 Nanotubes for Wide-Range Hydrogen Sensing. Nanoscale Research Letters, 9, p. 118. doi.org/10.1186/1556-276X-9-118.
- Galstyan, V., et al. (2013) TiO2 Nanotubes: Recent Advances in Synthesis and Gas Sensing Properties. Sensors, 13(11), pp. 14813–14838. doi.org/10.3390/s131114813.
- Ratautaite, V., et al. (2019) An Application of Conducting Polymer Polypyrrole for the Design of Electrochromic PH and CO2 Sensors. Journal of The Electrochemical Society, 166(6), pp. B297–B303. doi.org/10.1149/2.1221904jes.
- Celiesiute, R., et al. (2019) Electrochromic Sensors Based on Conducting Polymers, Metal Oxides, and Coordination Complexes. Critical Reviews in Analytical Chemistry, 49(3), pp. 195–208. https://doi.org/10.1080/10408347.2018.1499009
- Popov, A., et al. (2019) Comparative Study of Polyaniline (PANI), Poly(3,4-Ethylenedioxythiophene) (PEDOT) and PANI-PEDOT Films Electrochemically Deposited on Transparent Indium Thin Oxide Based Electrodes. Polymers (Guildf), 172, pp. 133–141. doi.org/10.1016/j.polymer.2019.03.059
- Turemis, M., et al. (2020) ZnO/Polyaniline Composite Based Photoluminescence Sensor for the Determination of Acetic Acid Vapor. Talanta, 211, p. 120658. doi.org/10.1016/j.talanta.2019.120658.
- Miller, D. R., et al. (2014) Nanoscale Metal Oxide-Based Heterojunctions for Gas Sensing: A Review.Sensors and Actuators B: Chemical, 204, pp. 250–272. doi.org/10.1016/j.snb.2014.07.074.
- Wu, Y., et al. (2010) Examining the Use of TiO2 to Enhance the NH3 Sensitivity of Polypyrrole Films. Journal of Applied Polymer Science, 118(6), pp. 3351–3356. doi.org/10.1002/app.32382.
- Tai, H., et al. (2007) International Journal of Environmental Analytical Chemistry Self-Assembly of TiO2/Polypyrrole Nanocomposite Ultrathin Films and Application for an NH3Gas Sensor. International Journal of Environmental Analytical Chemistry, 87(8), pp. 539–551. https://doi.org/10.1080/03067310701272954
- Bulakhe, R. N., et al. (2013) Fabrication and Performance of Polypyrrole (Ppy)/TiO2 Heterojunction for Room Temperature Operated LPG Sensor. Sensors and Actuators B: Chemical, 181, pp. 417–423. https://doi.org/10.1016/j.snb.2013.01.056
- Gong, J., et al. (2010) Ultrasensitive NH3 Gas Sensor from Polyaniline Nanograin Enchased TiO2 Fibers. The Journal of Physical Chemistry C, 114(21), pp. 9970–9974. doi.org/10.1021/jp100685r.
- Pawar, S. G., et al. (2012) Development of Nanostructured Polyaniline–Titanium Dioxide Gas Sensors for Ammonia Recognition. Journal of Applied Polymer Science, 125(2), pp. 1418–1424. doi.org/10.1002/app.35468.
- Wang, Q., et al. (2012) Ammonia Sensing Behaviors of TiO2-PANI/PA6 Composite Nanofibers. Sensors, 12(12), pp. 17046–17057. doi.org/10.3390/s121217046.
- Du, P., et al. (2012) Coaxial Electrospun TiO2/ZnO Core–Sheath Nanofibers Film: Novel Structure for Photoanode of Dye-Sensitized Solar Cells. Electrochim. Acta, 78, pp. 392–397. doi.org/10.1016/j.electacta.2012.06.034.
- Ding, Y., et al. (2011) Preparation of TiO2–Pt Hybrid Nanofibers and Their Application for Sensitive Hydrazine Detection. Nanoscale, 3(3), pp. 1149–1157. doi.org/10.1039/C0NR00773K.
- Li, Z., et al. (2008) Highly Sensitive and Stable Humidity Nanosensors Based on LiCl Doped TiO2 Electrospun Nanofibers. Journal of the American Chemical Society, 130(15), pp. 5036–5037. doi.org/10.1021/ja800176s.
- Zeng, W., et al. (2012) Enhanced Gas Sensing Properties by SnO2 Nanosphere Functionalized TiO2 Nanobelts. Journal of Materials Chemistry, 22(8), pp. 3544–3548. https://doi.org/10.1039/C2JM15017D
- Zakrzewska, K (2004) Gas Sensing Mechanism of TiO2-Based Thin Films. Vacuum, 74(2), pp. 335–338. doi.org/10.1016/j.vacuum.2003.12.152.
- Liang, Y. -C & Liu, Y -C (2019) Design of Nanoscaled Surface Morphology of TiO2–Ag2O Composite Nanorods through Sputtering Decoration Process and Their Low-Concentration NO2 Gas-Sensing Behaviors. Nanomaterials, 9(8), p. 1150. doi.org/10.3390/nano9081150.
- Wang, H., et al. (2014) A Micro Oxygen Sensor Based on a Nano Sol-Gel TiO2 Thin Film. Sensors, 14(9), pp. 16423–16433. doi.org/10.3390/s140916423.
- SA, M. C., et al. (2018) A Hydrogen Gas Sensor Based on TiO2 Nanoparticles on Alumina Substrate. Sensors, 18(8), 2483. doi.org/10.3390/s18082483.
- Park, S., et al. (2014) Effects of Functionalization of TiO2 Nanotube Array Sensors with Pd Nanoparticles on Their Selectivity. Sensors, 14(9), pp. 15849–15860. doi.org/10.3390/s140915849.
- Yavuz, A G & Gök, A (2007) Preparation of TiO2/PANI Composites in the Presence of Surfactants and Investigation of Electrical Properties. Synthetic Metals, 157(4–5), pp. 235–242. doi.org/10.1016/j.synthmet.2007.03.001.
- Oh, M., et al. (2012) Electrochemical Properties of Polyaniline Composite Electrodes Prepared by In-Situ Polymerization in Titanium Dioxide Dispersed Aqueous Solution. Synthetic Metals, 162(7–8), pp. 695–701. doi.org/10.1016/j.synthmet.2012.02.021.
- Guo, N., et al. (2014) Microscale Hierarchical Three-Dimensional Flowerlike TiO2/PANI Composite: Synthesis, Characterization, and Its Remarkable Photocatalytic Activity on Organic Dyes under UV-Light and Sunlight Irradiation. The Journal of Physical Chemistry C, 118(32), pp. 18343–18355. doi.org/10.1021/jp5044927.
- Gottam, R & Srinivasan, P (2015) One-Step Oxidation of Aniline by Peroxotitanium Acid to Polyaniline–Titanium Dioxide: A Highly Stable Electrode for a Supercapacitor. Journal of Applied Polymer Science, 132(13), p. 41711. doi.org/10.1002/app.41711.
- Radoičić, M., et al. (2010) Self-Assembled Polyaniline Nanotubes and Nanoribbons/Titanium Dioxide Nanocomposites. Synthetic Metals, 160(11–12), pp. 1325–1334. doi.org/10.1016/j.synthmet.2010.04.010.
- Su, S J & Kuramoto, N (2000) Processable Polyaniline–Titanium Dioxide Nanocomposites: Effect of Titanium Dioxide on the Conductivity. Synthetic Metals, 114(2), pp. 147–153. doi.org/10.1016/S0379-6779(00)00238-1
- Tai, H., et al. (2007) Fabrication and Gas Sensitivity of Polyaniline–Titanium Dioxide Nanocomposite Thin Film. Sensors and Actuators B: Chemical, 125(3), pp. 644–650. https://doi.org/10.1016/j.snb.2007.03.013
- Lee, I. S., et al. (2005) Synthesis and Electrorheological Characteristics of Polyaniline-Titanium Dioxide Hybrid Suspension. Synthetic Metals, 152(1–3), pp. 173–176. https://doi.org/10.1016/j.synthmet.2005.07.211
- Kang, K S (2016) Effect of Excess Amount of Aniline for TiO2 and Polyaniline Composite. Synthetic Metals, 217, pp. 197–201. doi.org/10.1016/j.synthmet.2016.03.026.
- Maldonado-Larios, L., et al. (2020) Electrochemically-Assisted Fabrication of Titanium-Dioxide/Polyaniline Nanocomposite Films for the Electroremediation of Congo Red in Aqueous Effluents. Synthetic Metals, 268, p. 116464. doi.org/10.1016/j.synthmet.2020.116464.
- Ilieva, M., et al. (2008) Electrochemical Synthesis and Characterization of TiO2-Polyaniline Composite Layers. Journal of Applied Electrochemistry, 38, pp. 63–69. doi.org/10.1007/s10800-007-9399-9
- Saeb, E & Asadpour-Zeynali, K (2021) Facile Synthesis of TiO2@PANI@Au Nanocomposite as an Electrochemical Sensor for Determination of Hydrazine. Microchemical Journal, 160(Part A), p. 105603. doi.org/10.1016/j.microc.2020.105603.
- Cai, G., et al. (2013) Multicolor Electrochromic Film Based on TiO2@Polyaniline Core/Shell Nanorod Array. The Journal of Physical Chemistry C, 117(31), pp. 15967–15975. doi.org/10.1021/jp4056939.
- Gobal, F & Faraji, M (2013) Electrodeposited Polyaniline on Pd-Loaded TiO2 Nanotubes as Active Material for Electrochemical Supercapacitor. Journal of Electroanalytical Chemistry, 691, pp. 51–56. doi.org/10.1016/j.jelechem.2012.12.008.
- Jagminas, A., et al. (2012) Electrochemical Synthesis and Characterisation of Polyaniline in TiO2 Nanotubes. Transactions of the IMF, 90(6), pp. 311–315. doi.org/10.1179/0020296712Z.00000000056.
- Patil, B. H., et al. (2017) Periodically Ordered Inverse Opal TiO2/Polyaniline Core/Shell Design for Electrochemical Energy Storage Applications. Journal of Alloys and Compounds, 694, pp. 111–118. doi.org/10.1016/j.jallcom.2016.09.331.
- Gao, L., et al. (2019) Facile Synthesis of the Composites of Polyaniline and TiO2 Nanoparticles Using Self-Assembly Method and Their Application in Gas Sensing. Nanomaterials, 9(4), p. 493. doi.org/10.3390/nano9040493.
- Ma, X., et al. (2006) Preparation of Polyaniline–TiO2 Composite Film with in Situ Polymerization Approach and Its Gas-Sensitivity at Room Temperature. Materials Chemistry and Physics, 98(2–3), pp. 241–247. doi.org/10.1016/j.matchemphys.2005.09.027.
- Huyen, D. N., et al. (2011) Effect of TiO2 on the Gas Sensing Features of TiO2/PANi Nanocomposites. Sensors, 11(2), pp. 1924–1931. doi.org/10.3390/s110201924.
- Tai, H., et al. (2008) Influence of Polymerization Temperature on NH3 Response of PANI/TiO2 Thin Film Gas Sensor. Sensors and Actuators B: Chemical, 129(1), pp. 319–326. doi.org/10.1016/j.snb.2007.08.013
- Bairi, V. G., et al. (2015) Viswanathan, T. Ammonia Gas Sensing Behavior of Tanninsulfonic Acid Doped Polyaniline-TiO2 Composite. Sensors, 15(10), pp. 26415–26429. doi.org/10.3390/s151026415
- Pawar, S. G., et al. (2011) Room Temperature Ammonia Gas Sensor Based on Polyaniline-TiO $ _ {2} $ Nanocomposite. IEEE Sensors Journal, 11(12), pp. 3417–3423. doi.org/10.1109/JSEN.2011.2160392.
- Seif, A., et al. (2019) UV Enhanced Ammonia Gas Sensing Properties of PANI/TiO2Core-Shell Nanofibers. Sensors and Actuators B: Chemical, 298, p. 126906. doi.org/10.1016/j.snb.2019.126906.
- Cui, S., et al. (2015) Fabrication and Design of a Toxic Gas Sensor Based on Polyaniline/Titanium Dioxide Nanocomposite Film by Layer-by-Layer Self-Assembly. RSC Advances, 5(72), pp. 58211–58219. doi.org/10.1039/C5RA06388D.
- Zhu, C., et al. (2018) Enhanced Sub-Ppm NH3 Gas Sensing Performance of PANI/TiO2 Nanocomposites at Room Temperature. Frontiers in Chemistry, 6, p. 493. doi.org/10.3389/fchem.2018.00493.
- Li, Y., et al. (2011) Fabrication of Polyaniline/Titanium Dioxide Composite Nanofibers for Gas Sensing Application. Materials Chemistry and Physics, 129(1–2), pp. 477–482. doi.org/10.1016/j.matchemphys.2011.04.045.
- Nasirian, S & Milani Moghaddam, H (2014) Effect of Different Titania Phases on the Hydrogen Gas Sensing Features of Polyaniline/TiO2 Nanocomposite. Polymer (Guildf), 55(7), pp. 1866–1874. doi.org/10.1016/j.polymer.2014.02.030.
- Gawri, I., et al. (2018) Chemically Synthesized TiO2 and PANI/TiO2 Thin Films for Ethanol Sensing Applications. Materials Research Express, 5(2), p. 025303. doi.org/10.1088/2053-1591/aaa9f1
- Wang, Z., et al. (2017) CO Gas Sensitivity and Its Oxidation over TiO2 Modified by PANI under UV Irradiation at Room Temperature. Applied Catalysis B: Environmental, 219, pp. 379–390. doi.org/10.1016/j.apcatb.2017.07.080
- Su, P G & Huang, L N (2007) Humidity Sensors Based on TiO2 Nanoparticles/Polypyrrole Composite Thin Films. Sensors and Actuators B: Chemical, 123(1), pp. 501–507. doi.org/10.1016/j.snb.2006.09.052.
- Srivastava, S., et al. (2011) Synthesis and Characterization of TiO2 Doped Polyaniline Composites for Hydrogen Gas Sensing. International Journal of Hydrogen Energy, 36(10), pp. 6343–6355. doi.org/10.1016/j.ijhydene.2011.01.141.
- Dhawale, D. S., et al. (2008) Room Temperature Liquefied Petroleum Gas (LPG) Sensor Based on p-Polyaniline/n-TiO2 Heterojunction. Sensors and Actuators B: Chemical, 134(2), pp. 988–992. doi.org/10.1016/j.snb.2008.07.003.
- Parveen, A., et al. (2013) Liquefied Petroleum Gas Sensing of Polyaniline–Titanium Dioxide Nanocomposites. Sensor Letters, 11(2), pp. 242–248. doi.org/10.1166/sl.2013.2732.
- Zheng, J., et al. (2008) Polyaniline–TiO2 Nano-Composite-Based Trimethylamine QCM Sensor and Its Thermal Behavior Studies. Sensors and Actuators B: Chemical, 133(2), pp. 374–380. doi.org/10.1016/j.snb.2008.02.037.